植物如何应对高山胁迫?

在高海拔地区,植物可能会受到强烈的胁迫:光照和紫外线辐射强度高,二氧化碳浓度低,昼夜温差大……这些参数随季节变化,但在几天之内或者同一天内也会发生显著变化。生活在高山环境中的植物利用光提供的能量进行光合作用得以生长发育,而光照、二氧化碳等限制性因子会调节或干扰植物的光合作用。尤其是,过量的光能会导致有毒化合物的合成,如活性氧(ROS)。为了适应这些困境,高山植物进化出了各种策略:非常小的体形、抵御紫外线辐射的保护屏障、保护性的解剖结构、耗散多余光能的机制、对活性氧的毒性消解等。
1.高山植被

在山区,从山谷到山顶,植被具有独特的地带性。在高山地区,低处是落叶林,到1800—2300米时,落叶林逐渐被针叶林所取代,具体取决于针叶林在何处出现取决于该地光照度(即阳面还是阴面):覆盖有一片疏林、其上为孤立树木的是亚高山层。再高一些,大约3000米处,是被草坪覆盖的斜坡,此处为高山层。高山层之上,破碎化植被(以被岩石、碎石等分隔的植被区域为特征。)、苔藓、地衣、裸岩和终年积雪替代了高山草坪,此处为积雪层[1],[2](图1)。在高海拔地段,植物多样性急剧下降[3],这时,气候因素似乎是大多数植物生长的限制性因素。
2.气候条件和生长季
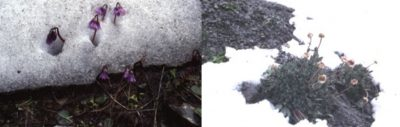
因此,多年生植物的优势是在生长季节只形成少数器官,而一年生植物必须形成它们所有的器官,这会使得植物在有限的时间内消耗大量的能量。

3.光合作用
在生长季,植物通过光合作用来保障自身的生长和发育,在这个过程中,太阳辐射提供必需的能量,才使得植物能够利用大气中吸收到的二氧化碳、水和土壤中的矿物质,合成有机物质。由于光合作用被这些高海拔地区存在的多种限制因子所影响[5],也许,能否在胁迫条件下保持光合作用,决定了植物能否在高海拔地区存活。光合作用的主要反应过程如图4所示,详细情况见[6]。图4还描述了高山植物为维持这一光合过程而具有的特性。

- 原初反应阶段发生在类囊体薄膜上:光能被光合色素(聚光叶绿素)吸收,随后转移到两个反应中心(光系统I和光系统II:PSI 和PSII),其协同活性通过一系列氧化还原反应,在PSI中将NADP+还原为NADPH(是辅酶烟酰胺腺嘌呤二核苷酸磷酸(NADP)的还原型。NADP 由烟酰胺腺嘌呤二核苷酸(或 NAD)通过与腺嘌呤相关核糖的 2’羟基结合形成。它以氧化态(NADP+)和还原态(NADPH)存在。据说,NADPH 具有还原能力:在氧化还原酶进行的催化反应中,它能够在氢原子转移过程中提供能量,从而进行细胞功能所需的还原反应。),还原所需要的电子来自于PSII中水的氧化反应(2H2O → O2+ 4H+ + 4e–)。原初反应阶段也诱导在类囊体膜上建立质子梯度,为ATP(三磷酸腺苷的缩写。一种三磷酸核苷,由腺嘌呤(氮基)、核糖(含5个碳原子的糖)和三个磷酸基团组成一个三磷酸基团。所有生物体中都存在的一种既能提供能量又能储存能量的化合物。也可用作核酸合成的原料。)的合成提供必要的能量(图4)。
- 光合作用的第二阶段发生在叶绿体基质中。它通过消耗ATP和NADPH来固定大气中的二氧化碳到由5个碳原子(二磷酸核酮糖,RuBP)作为骨架形成的二磷酸糖中(RuBisCO活性(1,5-二磷酸核酮糖羧化酶/加氧酶的缩写。 它是利用光合作用过程中叶绿素捕获的太阳能启动本森和卡尔文循环来固定植物生物质中二氧化碳的关键酶。)),从而产生含有三个碳原子的磷酸糖(磷酸三糖,简称TP)(图4)。这种迅速形成三碳化合物的光合作用过程,是C3植物(实行C3途径的植物)的特征。高山植物绝大多数为此类植物。
吸收了二氧化碳而形成的磷酸三糖(TP),在叶绿素细胞内有多种用途:(1)重新形成二氧化碳受体;(2)用于叶绿体中葡萄糖和淀粉的合成;(3)被输送到细胞的其他细胞器中,为它们的运作提供能量和碳链;(4)用于合成蔗糖,并输送到植物的其他部分,提供能量和碳骨架,以合成大量化合物[6]。还需要注意的是,氧气可以与二磷酸核酮糖结合:这种氧化过程是代谢途径和光呼吸的起始,光呼吸也同样消耗ATP和NADPH(图4)。
4.环境因素在光合作用中的重要性
4.1.温度
在光合作用过程中,温度主要影响二氧化碳和氧气固定,以及糖合成的反应,但也影响细胞间分子的交换。光合作用的两个主要阶段以及所涉及到的运输过程受温度的影响不同:
- 叶绿素色素吸收光、NADPH和ATP形成等生物物理过程,并不依赖或者是仅轻微依赖于温度。
- 二氧化碳和氧气吸收、糖合成这类生化反应,以及细胞间的分子交换,都高度依赖温度[7]。一般而言,增温10摄氏度会使得生化反应速率加倍。

例如,冰山金凤花(glacier buttercup),在23°C时、2000µmol photons m-2 s-1的光照下可以固定大概15µmolCO2 m-2 s-1(光通量数量级见图5),而其在10°C时只固定一半的二氧化碳。第一种情况下,光照不足,二氧化碳的固定足以抵消光照带来的能量,然而,在第二种情况下,二氧化碳的固定在500µmolphotons m-2 s-1下已经饱和,因此光基本上是过量的[8]。
4.2.光照
和温度一样,在高山环境下,光照可以在同一天突然变化几个数量级(图5)。在多云的天气下,山谷和高海拔地区[3]的平均光强差异不是很大。另一方面,在晴朗天气下,高海拔地区的最大光强度要大得多,超过了大多数植物的光合能力。就像温度骤降一样,极强光照会导致叶子中的能量过剩,从而损害光系统[7]。
此外,较高海拔地区的紫外线辐射(UVA和UVB)更强[9],这直接影响细胞结构:光系统,以及叶绿体、线粒体和细胞核中的DNA(参见“太阳紫外线对细胞的影响”)。在PSII中,参与产氧的锰(图4)和在PSII和细胞色素b6f(cytb6f)之间运输电子的质体醌,会直接吸收紫外线辐射,当紫外线辐射过高时,这些功能可能会受到抑制[10]。
阴生植物利用高山带常有的中强光,但如果光照强度过高,就会受到胁迫。另一方面,阳生植物对高强光更耐受,但对弱光的利用率很低[11]。此外,艳丽的高山植物,如高山雪铃花(Soldanella alpina)、山雏菊(Homogyne alpina )或毛茛宿萼(Ranunculus glacialis)等(图2),对弱光或强光没有表现出任何典型的特异性适应[12]。
4.3.其他影响因子
影响光合活性的其他高山环境因子:
然而,为应对水分胁迫,植物会关闭其气孔(控制叶片气体交换的特殊细胞):这限制了二氧化碳的供应,并减缓了光合作用。同样,光合作用的生化反应也不能消耗植物接收到的多余光能。
综上所述,山区的气候因子(如光照、温度、湿度)变化强烈、迅速,变化幅度较大。大多数平原植物在这种变化中是无法存活的,因为光合作用无法利用过剩的能量[7]。这些过剩的能量可以通过形成活性氧(ROS)转移到氧气中(图4,[12])。活性氧(ROS)是具有极强潜在破坏性的分子,它们不仅会损害光合作用装置,特别是光系统II(光抑制系统),还会损害到整个细胞(参见“植物的生长年限及其限制因子”)。最终,植物的光合能力可能会受到活性氧(ROS)的抑制,其修复损伤的能力也会大大降低[11]。
4.4. 高山植物光合作用的限制条件是什么?
高山环境所施加的胁迫可以用以下两种极端情况进行说明。一些高山植物,如高山雪铃花、水杨梅和高山良姜等,在冬季保留会一些绿叶。在高光强度和高热梯度相结合的条件下,这些叶片在雪融后立即开始进行光合作用[7]。这些高山植物会在融雪后的短时间内进行光合作用,因为几周后它们就会被其他植物遮蔽。在极端气候下,光合作用失活对这些植物可能是致命的。然而,大多数高山植物在冬天不保留叶子,必须快速利用所储存的能量去形成它的第一片叶子,确保它们在短周期内的发育。以毛茛宿萼(Ranunculus glacialis)(见图2)为例,我们仅在2200米以上发现毛茛宿萼(Ranunculus glacialis),这表明它的生存过程可能更加复杂。事实上,在这种植物中,据计算,形成叶片所调动的储备大约相当于30天的光合作用,这在生长季节很难实现(见图2)。由于毛茛宿萼(Ranunculus glacialis)需要两年的时间才能成熟[12],这表明在一个生长季节获得的能量不足以支撑其在一年内完成发育周期。因此,毛茛宿萼(Ranunculus glacialis)不存在光合作用失活的风险。一些高山物种的矮化现象也可以被认为是限制光合作用组织形成能量投资的一种策略。
5.高山植物的适应性
植物的适应性使其对环境的影响作出反应,在细胞和分子被影响到之前,减少环境引起的生理指标变化的幅度。当这些反应不足以缓解这些消极影响时,植物的适应性就通过其他保护措施表现出来,以防止细胞或其细胞器被破坏。
高山植物通常表现为垫状(参见图3)、莲座状或簇状特殊结构(参见“遗传或趋同作用”),这种形态使得植物生活在一个有利的小气候[3]中,如:(1)叶片组织所吸收的光强和紫外辐射减少;(2)叶片湿度增加,防风,避免干燥;(3)比起周边空气,植物温度变化更小。这种形态的形成代表了其具有抗逆性。当然,这些植物比不形成这些结构植物的生长速度更慢,这是高山植物为此需要付出的代价。
如上所述,极大热变化与高光照度能诱导光系统II的光抑制和活性氧(ROS)的形成。
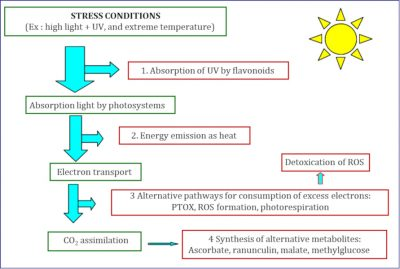
(图6 STRESS CONDITIONS胁迫条件;Ex: high light+UV, and extreme temperature例:强光+紫外线,极端温度;1.Absorption of UV by flavonoids通过类黄酮吸收紫外线;Absorption light by photosystems通过光合系统吸收光;Energy emission as heat以热能的形式释放能量;2.ectron transport电子传递;Detoxication of ROS活性氧的解毒;3.Alternative pathways for consumption of excess electrons: PTOX, ROS formation, photorespiration 多种消耗过剩电子的途径:质体末端氧化酶,活性氧,光呼吸;CO2 assimilation吸收二氧化碳;4.Synthesis of alternative metabolites: Ascorbate, ranunculin, malate, methylglucose 合成多种代谢产物:抗坏血酸,毛茛甙,苹果酸,甲基葡萄糖)
与低地植物相比,我们研究的高山植物[7]对光抑制具有更强的抗性。为此,高山植物采用了不同的保护机制,这些机制大多数也存在于低地植物中。这些是发生在多个组织层级的回避机制(多种保护机制的详细信息见图4)。它们可以分为两类:“上游”机制,避免能量到达反应中心的速度过快;“下游”机制,即使在不利条件下,也要保持光合反应中所分离出电子的利用(即充分使用聚光色素所捕获的光能)(见图6)。
- 防止紫外线辐射。在植物的表皮层,一些物种能够合成植物的此生代谢产物——黄酮类物质,这类物质都有相同的基本结构。黄酮类化合物是多酚类化合物中最重要的一类,是一个庞大的抗氧化剂家族。黄酮类化合物是植物花、果产生棕色、红色和蓝色色素的原因,这些色素可以吸收高能的紫外辐射。因此,紫外线不会刺激到下层细胞。因此,这种类黄酮屏障可以保护光系统,防止含叶绿素的薄壁细胞的DNA遭到破坏。叶绿体作为光合作用的场所,它们参与植物的营养功能。它们也是叶片内部的重要组成部分。以高山雪玲花为例,高强度光照会诱导其类黄酮含量上升,从而保护植物,且这种保护的力度随着海拔的升高而增加[13]。
- 所吸收的过剩能量的再发射。光照植物聚光色素构象变化;与此同时,一些被激活的叶绿素将其能量传递给类胡萝卜素(例如玉米黄素)。这使得一些光能以热量的形式耗散(图4)。这样,到达反应中心的能量就被控制了。在过量的光照下,热耗散的光能量高于直接传到反应中心的光能量,热耗散是一种去除叶绿素激发态的快速方法。在一些像高山雪铃花和水杨梅花这类高山植物中,这种回避机制非常有效,而在其他像毛莨宿萼(Ranunculus glacialis)这类物种中,这种机制就不是那么有效了,而是激活其他机制(见下文)[7]。
- 能量通过电子流转移到替代受体。在叶绿体中,连接两个光系统的替代氧化酶(称为PTOX)(叶绿体类囊体膜的酶,催化质体醌库的氧化,因此得名替代氧化酶(PTOX)。)(图4),接受PSII提供的电子,并将其转移到氧分子中,从而形成水。因此,这种酶可以降低电子压力,减少因过量光照而形成的活性氧(ROS)。与低地植物相比,高山植物内替代氧化酶含量高,且其浓度随着海拔的升高而增加。
- 在低温下维持光合代谢。如果二氧化碳的供应限制了光合作用第二阶段糖的合成,Rubisco酶会催化分子氧与二磷酸核酮糖的结合。这是光呼吸系统的第一步(图4)。光呼吸作用消耗能量,其形式包括(a)碳(二磷酸核酮糖)、(b)光合作用第一阶段产生的电子和(c)ATP。在低温下,光呼吸作用通常可以忽略不计,但当温度超过20摄氏度时,光呼吸作用就会很重要。对于不耐热的毛茛宿萼(Ranunculus glacialis)来说,温度很低时,其光呼吸作用就已经很活跃了。
- 特定代谢产物的合成。一些高山植物合成的代谢物在低地植物中通常不存在或少量存在。这些代谢物的功能往往尚不清楚。但这些代谢物的合成会消耗光合作用装置所捕获的部分能量,从而可以帮助保护光合作用装置。因此,毛莨会积累大量的毛莨苷和苹果酸[14](苹果酸盐是一种广泛应用于植物界的二羧酸,天然存在于水果中,使水果味道宜人。苹果酸是克雷布斯循环的中间体,克雷布斯循环是几乎所有生物细胞呼吸的主要代谢途径之一,苹果酸还参与光合作用中的本森和卡尔文循环。用作食品添加剂,编号为 E296)[14]。水杨梅含有高浓度的甲基葡萄糖,而高山雪铃花的叶片[15]中含有创纪录数量的抗坏血酸(维生素C)。抗坏血酸是抗氧化系统的一部分,可以保护植物免受活性氧(ROS)的侵害。
- 高活性氧(ROS)的解毒。环境胁迫增加了活性氧(ROS)的形成,特别是在光合作用的原初反应阶段。如图4所示,活性氧(ROS)的形成显示在PSI水平上:它是在光照下活性氧(ROS)形成的主要途径。为了将活性氧(ROS)降解为氧气,植物会进行一系列与代谢产物相关的酶反应。在高山雪铃花的叶片中,维生素C、维生素E和参与氧化还原过程的酶是抗氧化系统所必需的。在委陵莱中,叶片抗氧化系统的重要性随着海拔的升高而增加[16]。
简而言之,正是这样一套保护机制,不仅可以让高山植物存活下来,而且可以使其在随着海拔升高、限制作用日益增强的栖息地生活。这些保护机制相互补充,帮助植物在高山环境中生存下来(图6)。
参考资料和说明
封面照片: 加利西亚岩层上的高山柳穿鱼(Linaria alpina)(2600m处)。 [来自: 照片©谢尔盖·奥伯特(Serge Aubert)/阿尔卑斯山约瑟夫傅里叶站(SAJF)]
[1] Ozenda P., La végétation de la chaîne alpine dans l’espace montagnard européen, Masson, 1985
[2] Fischesser B., La vie de la montagne, Éditions de La Martinière, Paris, 1998
[3] Körner C., Alpine plant life, Springer Verlag, Berlin Heidelberg, 1999
[4] Larcher W., Kainmüller C., Wagner J., Survival types of high mountain plants under extreme temperatures, Flora, 205:3-18, 2010
[5] Lütz C., Plants in Alpine Regions, Cell Physiology of Adaptation and Survival Strategies, Springer, Wien New York, 75-97, 2012
[6] Raven P., Evert R., Eichhorn S., Biology of Plants, Sixth Edition, W.H. Freemann and Company/Worth Publishers, 1986
[7] Streb P., Cornic G., Photosynthesis and Antioxidative Protection in Alpine Herbs, In Lütz C. (Ed) Plants in Alpine Regions: Cell Physiology of Adaptation and Survival Strategies, Springer Wien New York, pp 75-97, 2012
[8] Streb P., Josse E.-M., Gallouët E., Baptist F., Kuntz M., Cornic G. (2005) Evidence for alternative electron sinks to photosynthetic carbon assimilation in the high mountain plant species Ranunculus glacialis. Plant, Cell Environment 28:1123-1135
[9] Barry R., Mountain weather and climate, Cambridge University Press, Third Edition, 2008
[10] Teramura A., Ziska L., Ultraviolet-B radiation and photosynthesis, In “Advances in Photosynthesis Vol. 5: Photosynthesis and the Environment“. Ed. N.R. Baker, pp 435-450, Kluwer Academic Publishers, Dordrecht, 1996
[11] Walters R., Towards an understanding of photosynthetic acclimation, Journal of Experimental Botany 56:435-447, 2005
[12] Ort D., Baker N., A photoprotective role of O2 as an alternative electron sink in photosynthesis? Current Opinion in Plant Biology 5:193-198, 2002
[13] Laureau C., Meyer S., Baudin X., Huignard C., Streb P. (2015) In vivo epidermal UV-A absorbance is induced by sunlight and protects Soldanella alpina leaves from photoinhibition. Functional Plant Biology, 42:599-608
[14] Streb P., Aubert S., Gout E., Bligny R. (2003) Reversibility of cold- and light-stress tolerance and accompanying changes of metabolite and antioxidant levels in the two high mountain plant species Soldanella alpina and Ranunculus glacialis. J. Exp. Bot. 54:405-418
[15] Bligny R., Aubert S., Specifities of metabolite profiles in alpine plants. In Lütz C. (Ed) Plants in Alpine Regions: Cell Physiology of Adaptation and Survival Strategies, Springer, Wien & New York, pp 99-120, 2012
[16] Lan Ma, Xudong Sun, Xiangxiang Kong, Jose Valero Galvan, Xiong Li, Shihai Yang, Yunqiang Yang, Yongping Yang, Xiangyang Hu, Physiological, biochemical and proteomics analysis reveals the adaptation strategies of the alpine plant Potentilla saundersiana at altitude gradient of the Northwestern Tibetan Plateau, Journal of Proteomics, 112:63-82, 2015
环境百科全书由环境和能源百科全书协会出版 (www.a3e.fr),该协会与格勒诺布尔阿尔卑斯大学和格勒诺布尔INP有合同关系,并由法国科学院赞助。
引用这篇文章: STREB Peter, CORNIC Gabriel, BLIGNY† Richard (2024年3月12日), 植物如何应对高山胁迫?, 环境百科全书,咨询于 2025年4月24日 [在线ISSN 2555-0950]网址: https://www.encyclopedie-environnement.org/zh/vivant-zh/how-do-plants-cope-with-alpine-stress/.
环境百科全书中的文章是根据知识共享BY-NC-SA许可条款提供的,该许可授权复制的条件是:引用来源,不作商业使用,共享相同的初始条件,并且在每次重复使用或分发时复制知识共享BY-NC-SA许可声明。