Plant resistance to stress: role of respiration?
PDF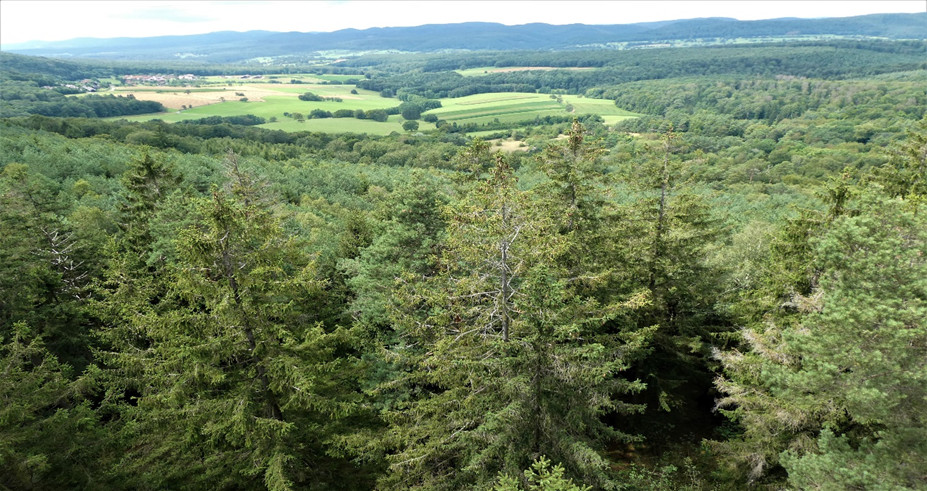
Yes, plants breathe! Their growth is the result of a delicate balance between the photosynthetic acquisition of carbon and the respiratory restitution of part of it. During photosynthesis, plants absorb carbon dioxide, CO2, whose increasing presence in the atmosphere is a problem, and release oxygen, O2. This gas exchange characterizes the autotrophy of plants, i.e. their ability to manufacture organic compounds from water, minerals and CO2 in the presence of light. During respiration, plants degrade part of the compounds formed by photosynthesis and produce energy; they then consume oxygen and release CO2. This is how the plant cell synthesizes the many compounds that make up its biomass. But what happens in case of stress? How do the respiratory mechanisms allow the synthesis of defence compounds, and do they then contribute to an adapted response of the plants to these stresses?
1. Gas exchange in plants
The photosynthesis of a plant concerns only the aerial chlorophyllous parts, i.e. mainly the leaves and the green stems, when the plant is young.
On the contrary, respiration concerns the whole plant. It is the equivalent of a combustion reaction -it uses dioxygen and releases carbon dioxide- which allows to transform carbon compounds like glucose into energy. The underground parts, i.e. the roots, also breathe. There is indeed an atmosphere in the soil which, in a well-drained environment, contains about 19% O2 (21% in the atmosphere), 79% nitrogen N2 (78% in the atmosphere) and 0.3% CO2 (0.04% in the atmosphere), conditions that are favourable to the respiratory process. The respiration of the aerial parts, concerns leaves and stems, without forgetting fruits and seeds.
For an annual plant, the gas exchanges are thus distributed between:
- Photosynthesis, which takes place exclusively during the day in the aerial parts. It is dominant but not exclusive since an aerial respiration co-exists.
- Subterranean respiration, which is essential since its role is to degrade sugars (mainly sucrose) transmitted via the elaborated sap from the leaves to the roots.
This does not obviate the interest of leaf respiration, which contributes to their cellular and tissue life (see paragraph 2). Respiration takes place day and night, unlike photosynthesis.
Table 1. Gas exchange of the different organs of a plant. PG: Gross photosynthesis; RD: Day respiration; RN : Night respiration; PR: Photorespiration.
- Photosynthesis (PB) takes place only during the day in the light and exclusively mobilizes chloroplasts.
- Respiration takes place day and night and mobilizes the degradative processes of the cytosol (glycolysis, pentose phosphate pathway) and mitochondria. However, day (RD) and night (RN) respiration can show different intensities (see paragraph 2.1).
- Photorespiration (PR) takes place only in the light and involves chloroplasts, peroxisomes and mitochondria (Figure 1, See The Path of Carbon in Photosynthesis) (see paragraph 2.2). Since photorespiration works at best at 21% O2, it is likely to be very low in green stems that contain a lower O2/CO2 ratio than in the atmosphere.
Figure 2 shows an example of gas exchange measurements (CO2 absorption or emission) of young vine plants [1] :
- During the daytime (diurnal period), the curve represents net photosynthesis (PN); photosynthetic processes dominate over respiratory processes.
- During the dark period, only respiration operates. Night respiration (RN) then represents barely 20% of net photosynthesis (PN), and an even smaller percentage compared to gross photosynthesis (PG), if we take into account the respiratory phenomena that take place during the day (dayrespiration RD and photorespiration PR), which we will discuss later (see paragraph 2).
- Curve 1 represents the gross photosynthesis (PG) of the aerial parts.
- Considering the respiration (RD) of the leaves gives curve 2, which is lower than curve 1.
- Further subtraction of leaf losses results in the net photosynthesis (PN) of the tree in curve 3.
- Curve 4 considers the respiration of the trunk, branches and roots.
- Finally, the net gain (curve 5) is obtained by subtracting the losses of branches and roots: it is the lowest curve that reflects the total balance.
Figure 2 shows that the carbon gain by a tree like the beech is very important in its youth (up to 20 years), and then reaches a plateau [2]. This mode of operation can be altered by environmental constraints.
2. The role of respiration in leaf gas exchange
2.1. Gas exchange balance
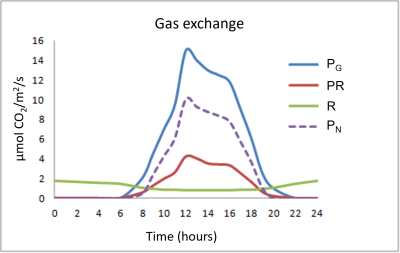
Figure 4 summarizes the different gas exchanges taking place in leaves during a 24-hour cycle:
- The chloroplastic photosynthetic process (gross photosynthesis PG) takes place during the day and assimilates CO2 while releasing O2.
- Respiration (R), on the other hand, consumes O2 and rejects CO2, and, again unlike photosynthesis, takes place at night and day. Respiration is often referred to as mitochondrial respiration because the mitochondria are at the heart of this process. It is also important to note that day respiration (RD) is weaker than night respiration (NR).
- Finally, a rather particular process is added to the two previous ones: photorespiration (PR) which takes place only during the day, consumes O2 and rejects CO2
Photorespiration represents about 20 to 25% of gross photosynthesis in C3 plants. Regarding respiration, the nocturnal respiratory intensity (RN) reaches 10 to 15% of gross photosynthesis.
Net photosynthesis (PN), measured during the diurnal phase, is represented by the formula:
- PN = PG – (PR + RD)
and could be written:
- PN = PG – (0.25 PG + 0.05 PB) or PN = PB – 0.3 PB
which means that the “respiratory” type processes, causing O2 absorption and CO2 release, reduce photosynthesis by 30% of its efficiency.
2.2. Photorespiration or photorespiratory cycle
- By its carboxylase function, RubisCO integrates a CO2 molecule on a ribulose bisphosphate molecule (C5 molecule) and produces carbon compounds with 3 carbon atoms (C3): phosphoglyceric acid (APG) and trioses phosphates (PGAL).
- Through its oxygenase function, RubisCO integrates an O2 molecule to the ribulose bisphosphate molecule (C5 molecule) which is cut into APG (C3 molecule) and phosphoglycolate (carbon compound with two carbon atoms, C2). APG can join the pool of APG from the carboxylase function while the formation of phosphoglycolate marks the beginning of the photorespiratory cycle.
This cycle sees (i) a succession of C2 compounds leaving the chloroplast and (ii) crossing the peroxisome before being (iii) transformed into a C2 amino acid, glycine, in the mitochondria. It is (iv) in the mitochondria that CO2 will be released (as well as NH3 ammonia) in a complex enzymatic reaction that will give serine, a C3 amino acid. The (v) return to the chloroplast (hence the name of cycle) then takes place by (vi) crossing the peroxisome again, finally giving (vii) an APG which can further increase the pool of these compounds within the chloroplast.
This succession of biochemical reactions:
- is globally energy consuming (ATP).
- does not assimilate CO2but on the contrary leads to a net loss of carbon by CO2 emission.
It should also be noted that additional O2 is consumed in the peroxisome during the oxidation of glycolate. For completeness, the NADH from the operation of glycine decarboxylase, a member of the enzyme complex responsible for the conversion of glycine to serine, can be re-oxidized by the mitochondrial respiratory chain, leading to even more oxygen consumption (see also paragraph 2.3).
2.3. Mitochondrial respiration
2.3.1. Supply of carbon compounds to the mitochondria
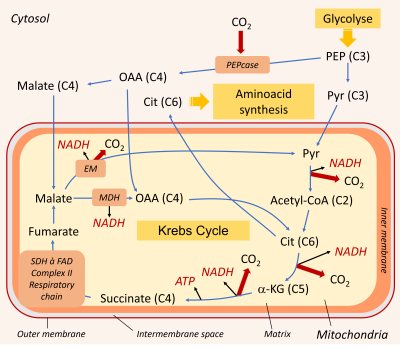
Mitochondrial respiration requires a supply of carbon compounds from cytosolic sugar degradation pathways to the organelle. Two pathways share this task: glycolysis and the hexose (C6 sugars) monophosphate pathway (HMP). At the end of the chain, glycolysis yields phosphoenolpyruvate (PEP) and pyruvate (Pyr) (Figure 6):
- Pyruvate (at C3) is one of the three main organic acids to enter the mitochondria.
- PEP (in C3) allows to obtain the other two(oxaloacetate and malate, organic acids in C4) by a sequence of two enzymatic reactions taking place in the cytosol. The phosphoenolpyruvate carboxylase (PEPcase) allows to obtain, by carboxylation, oxaloacetate (C4) which then gives malate (C4).
Mitochondrial respiration itself is divided into two parts:
- The Krebs cycle, which takes place in the mitochondrial matrix.
- The respiratory chain, which is integrated into the inner membrane of the mitochondria.
2.3.2. The Krebs cycle
The Krebs cycle is fed by the three organic acids described above (pyruvate, oxaloacetate and malate). It consists of a succession of enzymatic reactions, some of which release CO2 and deliver energy products in the form of ATP and NADH:
- One of the enzymes involved in the Krebs cycle, succinate dehydrogenase (SDH) is integrated into the respiratory chain in complex II.
- During the oxidation of succinate to fumarate, the co-enzyme FAD is reduced to FADH2 (Figure 7).
- This FADH2, as well as NADH, is then re-oxidized by the respiratory chain.
- NADH is reduced either at the level of complex I, or through an NADH dehydrogenase enzyme located on the inner side of the mitochondrial inner membrane (NADin).
As for citrate, it can leave the mitochondria and serve as a precursor for the synthesis of amino compounds involved in stress resistance (Figure 6) (see paragraph 3.3).
2.3.3. The respiratory chain or electron transfer chain
The mitochondrial respiratory chain consists of:
- A series of respiratory complexes I, III and IV, linked together by electron and proton transporters, taking electrons supplied by NADH and bringing them to the final stage of reduction of dioxygen O2to H2O (Figure 7).
- Complex II, on the other hand, works with FADH2.
- The transporters involved are essentially cytochromes (small iron proteins) and ubiquinones (UQ, small molecules with a terpene chain and mobile within the lipid membrane) and the chain is often called the cytochromic electron transport pathway.
- During this pathway, protons are released into the intermembrane space three times, contributing to the establishment of a proton gradient.
- The return of protons into the matrix, channelled by an ATPase (or ATP synthase; see Focus ATP Synthesis), allows the synthesis of ATP (Figure 7).
Presence of an alternative oxidase (AOX). Plants are characterized by the existence of a cyanide-resistant respiration, particularly evident during thermogenesis in Araceae (see focus Thermogenesis and pollination in Araceae). This cyanide resistance corresponds to the functioning of a double mitochondrial electron transport pathway:
- The cytochromic pathway, sensitive to cyanide and coupled to the production of ATP.
- A cyanide-insensitive, non-phosphorylating pathway [3].
The existence of these two pathways allows modulation of ATP production according to cellular energy requirements [3].
The ubiquinone pool was identified as the branching point between the two electron transfer pathways [4]. The terminal compound of the cyanide-insensitive electron transfer pathway, dependent on nuclear protein synthesis [5], is a quinol oxidase, called AOX (for Alternative OXidase in English) [6].
The synthesis of alternative oxidase (AOX) is (i) controlled by the nucleus in a direct manner and (ii ) through signals from the mitochondria (retrograde signalling) and (iii ) its expression is regulated in a biochemical post-translational manner [7]. AOX can take over part of the electron transport while reducing overall ATP production. The contributions of the respiratory chain complexes and the two terminal oxidases (cytochrome oxidase and alternative oxidase) to electron transport will adjust the amount of ATP required for energy needs.
This plasticity allows the mitochondrial AOX to play a prominent role in the stress response of plants [8] (see section 3.4.2).
Presence of an uncoupling protein. Discovered in 1995 in plant mitochondria, the uncoupling protein (UCP):
- is inserted in the inner membrane of mitochondria.
- reduces the relationship between the proton gradient linked to electron flow and ATP synthesis [9] (Figure 8).
- allows protons released in the intermembrane space to flow back to the matrix.
- allows a strong electron transport without concomitant ATP synthesis, which can be interesting during stress (see paragraph 3).
3. Role of respiratory processes in the stress response
3.1. CO2 release: loss or necessity?
- Only one-third of the assimilative efforts of photosynthesis are dissipated during respiration (see paragraph 2).
- Respiratory processes are essential for the growth of the various plant tissues by providing three objectives:
- The utilization/transformation of the initial products of photosynthesis (mainly sugars) into carbon skeletons that will enable the synthesis of elaborate compounds.
- The production of cellular energy (in the form of ATP), essentially at the level of mitochondria, respiratory cellular organelles, necessary to the realization of these syntheses.
- The maintenance of a cellular redox balance by adjusting, still essentially at the mitochondrial level, the ratios between the reduced and oxidized forms of NADH and NADPH molecules as well as the production of ROS (reactive oxygen species) (See Environmental constraints and oxidative stress in plants).
In addition, an increase in respiratory intensity, sometimes transient, is commonly observed during stresses suffered by plants or during events requiring a significant mobilization of small carbon compounds. The phenomenon of thermogenesis is emblematic of the latter case [3] (See focus Thermogenesis and pollination in Araceae).
During environmental stress, the balance between energy production and the use of carbon compounds for growth is disturbed. Indeed, part of the intermediate carbon compounds are diverted to the production of secondary compounds to counteract the damage caused by the stress. But is an increase in respiratory intensity able to satisfy all the needs (growth and defence)?
3.2. Photorespiration
Photorespiration, which occurs exclusively during the day, plays a significant role in the response to environmental stresses.
- The initial stage of the process, which involves competition between the two functions (carboxylase and oxygenase) of RubisCO, is very sensitive to the ratio between the concentrations of CO2 and O2 in the immediate environment of the Rubisco. At least two stresses, both related to current changes in climatic components, are likely to increase the O2/CO2 ratio and thereby cause an increase in the photorespiratory cycle.
- An increase in temperature decreases both the specificity of RubisCO for CO2 and the solubility of the gas, leading to higher O2 pressure in the leaf cells.
- Drought, often linked to increased temperature, leads to stomatal closure, limiting CO2 entry and promoting oxygenase function, thus photorespiration. The reduction of CO2 assimilation, linked to the decrease of the carboxylase function, reduces the consumption of reducing compounds and ATP from the photosynthetic electron transport chain. However, the photosynthetic electron transport chain continues to capture light energy with a very high risk of excess electrons that can lead to the accumulation of ROS and NADPH.
- Photorespiration, by using, at least in part, the excess of electrons during some of its enzymatic reactions, protects the photosynthetic apparatus from photoinhibition. It is also able to replenish the Calvin-Benson-Bassham cycle with APG and CO2, delivered to the mitochondria during glycine oxidation (see Figure 5). Photorespiration could thus contribute to alleviate a detrimental accumulation of reduced equivalents, in parallel with specific transfer mechanisms for the removal of this excess reducing power (present in NADPH) from the chloroplast to the cytosol and mitochondria.
3.3. Cytosolic degradation pathways
- to the supply of more organic acids (phosphoenolpyruvate, oxaloacetate, malate, pyruvate; Figure 10) to the contact of the mitochondria.
- the removal of intermediate compounds along the degradative pathways towards the synthesis of secondary compounds linked to the defense against stress(flavonoids, phenylpropanoids…).
An interesting example is that of pyruvate, which is the emblematic 3-carbon compound of the final stage of glycolysis (Figure 10). Pyruvate, discovered by Berzelius in 1835 during the fermentation of grapes, derives etymologically from the combination of the Greek pyro (fire) and the Latin uva (grape). Pyro, because this molecule is burned (we should say oxidized) to produce energy. This pyruvate comes essentially from sugars but can be produced by degradation of lipids or proteins. Its production from sugars delivers 20 to 30% more CO2 than the other two sources [11].
Moreover, pyruvate is involved in the stimulation of AOX activity, which is an advantage in case of stress (see paragraph 3.4.2).
It should be added that citrate, the first organic acid formed in the Krebs cycle (see Figure 6), can be exported from the mitochondria to the cytosol to serve as a precursor for the synthesis of amino compounds involved in stress resistance [12].
3.4. Mitochondria and ATP production
Organic acids from the cytosol will be metabolized in the mitochondrial matrix in the Krebs cycle, also called the tricarboxylic acid cycle (see Figure 6). CO2 and reducing power (mainly in the form of NADH) are produced during this process. The reoxidation of the reduced compounds takes place in the presence of oxygen thanks to the respiratory chain inserted in the inner membrane of the mitochondria and allows the production of energy in the form of ATP (see Figure 7). But the functioning of this respiratory chain is inevitably accompanied by the production of Reactive Oxygen Species (ROS), a production that must be regulated, especially since stresses lead to an increased production of these ROS [12] (See Environmental constraints and oxidative stress in plants).
The mitochondria will therefore present a whole range of solutions to best respond to the stresses suffered by plants, at least during stresses of moderate intensity or duration.
3.4.1. The different electron transport pathways
The electrons supplied by reduced NADH-type compounds (sometimes also NADPH), produced in the mitochondrial matrix by the Krebs cycle and associated enzymes, are taken through a redox chain involving many electron and sometimes proton transporters, some of which are nested in complexes (I, III and IV) (see Figure 7).
- Complexes I, III and IV are indeed associated with proton emission into the intermembrane space.
- As for the electrons transmitted by FADH2, they are specifically taken up by complex II, which is not associated with proton transfer (Figure 7).
- Pathway 1 (in blue), the most energetic, passes through all the complexes and allows 3 ATP to be obtained.
- Pathway 2 (in purple) uses complex I but bypasses the other 2 complexes by using AOX, which leads to the production of 1 ATP
- Pathway 3 (green) short-circuits complex I using NDin, then uses complexes III and IV, resulting in 2 ATP.
- Pathway 4 (in red) bypasses all complexes via NDin and AOX, allowing no ATP production.
- As for the electrons coming from FADH2, their pathway can include the last two complexes III and IV, allowing to obtain 2 ATP, or use only AOX, leading to 0 ATP.
This plasticity is already an interesting asset allowing to modulate the amount of energy necessary for the harmonious functioning of plant cells in favourable environmental conditions:
- In case of stress, the distribution of electrons in the different pathways will be able to evolve to favour the combination adapted to the new energy demand.
- The production of ROS at the level of complexes I, II and III, which is usual under favourable conditions, systematically increases during stress, which makes it interesting to use pathways that favours transporters that short-circuit these complexes (see 3.4.2 and 3.4.3).
3.4.2. The alternative oxidase (AOX)
The alternative, so-called non-phosphorylating oxidase, especially in combination with the internal NADH dehydrogenase (NDin) which short-circuits complex I, considerably reduces ROS production. Its increased involvement in electron transport during stress has been demonstrated [8,12] (See Environmental stress and oxidative stress in plants).
The functioning of AOX is indeed stimulated by the presence of more abundant quantities of pyruvate during stress and effectors signal the nucleus to initiate the synthesis of the mitochondrial enzyme. The function of AOX in response to environmental stresses therefore seems particularly important (Figure 12) [13].
3.4.3. The uncoupling protein (UCP)
The importance of the uncoupler protein increases under stress because it prevents the overproduction of ATP [14]. The protons in the intermembrane space return to the matrix, bypassing ATP synthetase. The decrease of the membrane potential then strongly decreases the production of ROS without affecting the essential production of energy. The decoupling protein thus participates, together with AOX, in the reduction of the potentially toxic presence of these ROS in mitochondria and cells [15].
4. Messages to remember
- Respiration and photosynthesis are complementary processes involved in the balanced growth of plants. Therefore, misuse of the term “respiratory loss” should be avoided.
- Respiratory processes include photorespiration and respiration sensu stricto, sometimes called “mitochondrial”. Photorespiration takes place during the day and cyclically involves chloroplasts, peroxisomes and mitochondria. Respiration, on the other hand, takes place day and night, and involves degradative processes located in the cytosol (glycolysis and the pentose phosphate pathway) and the mitochondria.
- About one third of the photosynthetic assimilation of CO2 is used by respiratory processes. These processes essentially play a dual role of ATP production (total respiration until CO2 emission) and use of intermediate carbon compounds to carry out the synthesis of structural compounds necessary for growth.
- Environmental stresses result in an increase in respiratory processes with increased synthesis of carbonaceous compounds necessary for the defence of the plant. Added to a decrease in photosynthetic assimilation, this new distribution of available resources could lead, in the medium term, to an unfortunate imbalance for growth. Moreover, stresses cause an increase in the formation of reactive oxygen species (ROS).
- To reduce the risk of toxicity caused by too much ROS production, mechanisms are implemented to favour mitochondrial systems that weaken ATP production and reduce the use of ROS producing complexes (AOX, UCP, NDin).
- This only works if the stress is not too intense or is of short duration. Otherwise, the increasing depletion of carbon resources from assimilation, combined with an increasing demand for the supply of defence compounds, would inexorably lead to an increasing reduction in growth.
Notes & References
Cover image. Landscape taken from the path of the peaks towards Drachenbronn-Birlenbach, Vosges du Nord, Regional Nature Park [Source: Photo © P. Dizengremel, August 2021]
[1] Bediee A, Luchaire N, Dambreville A, Brichet N, Hamard P, Dauzat M, Muller B & Pellegrino A (2015). Serial measurements of whole-plant scale gas exchange of pot-grown plants. Cahier des Techniques de l’INRA, 86, 21 p. hal-01329223
[2] Möller CM, Müller D, & Nielsen J (1954). Graphic presentation of dry matter production of European beech. Det Forstlige Forsogsvoesen i Danmark, XXI, 327-335.
[3] Meeuse BJD (1975). Thermogenic respiration in aroids. Annu. Rev. Plant Physiol, 26, 117-126.
[4] Storey BT (1976). Respiratory chain of plant mitochondria. XVIII. Point of interaction of the alternative oxidase with the respiratory chain. Plant Physiol, 58, 521-525.
[5] Dizengremel P & Lance C (1976). Control of changes in mitochondrial activities during aging of potato slices. Plant Physiol, 58, 147-151.
[6] Hiser C & McIntosh L (1990). Alternative oxidase of potato is an integral membrane protein synthesized de novo during aging of tuber slices. Plant Physiol, 93, 312-318.
[7] Vanlerberghe GC (2013). Alternative oxidase: a mitochondrial respiratory pathway to maintain metabolic and signaling homeostasis during abiotic and biotic stress in plants. Int. J. Mol. Sci, 14, 6805-6847; doi:10.3390/ijms14046805.
[8] Selinski J, Scheibe R, Day D & Whelan J (2018). Alternative oxidase is positive for plant performance. Trends in Plant Science, 23, 588-597; https://doi.org/10.1016/j.tplants.2018.03.012.
[9] Vercesi AE, Borecký J, de Godoy Maia I, Arruda P, Cuccovia IM, & Chaimovich H (2006). Plant uncoupling mitochondrial proteins. Annu. Rev. Plant Biol. 57, 383-404. doi: 10.1146/annurev.arplant.57.032905.105335.
[10] Free Psikoda! Journal La Hulotte No. 26, 1 January 1985; https://www.lahulotte.fr/
[11] Le XH, Lee CP, Monachello D, & Millar AH (2022). Metabolic evidence for distinct pyruvate pools inside plant mitochondria. Nature Plants, 8, 694-705; https://doi.org/10.1038/s41477-022-01165-3.
[12] Gandin A, Dizengremel P, & Jolivet Y (2021). Integrative role of plant mitochondria facing oxidative stress: the case of ozone. Plant Physiol. Biochem. 159, 202-210. https://doi.org/10.1016/j.plaphy.2020.12.019.
[13] Saha B, Borovskii G, Kumar Panda S (2016). Alternative oxidase and plant stress tolerance. Plant Signaling and Behavior, 11, e1256530-1 – e1256530-4. http://dx.doi.org/10.1080/15592324.2016.1256530
[14] Barreto P, Counago RM, & Arruda P (2020). Mitochondrial uncoupling protein-dependent signaling in plant bioenergetics and stress response. Mitochondrion, 53, 109-120. https://doi.org/10.1016/j.mito.2020.05.001.
[15] Borecky J, & Vercesi AE (2005). Plant uncoupling mitochondrial protein and alternative oxidase: energy metabolism and stress. Bioscience Reports, 25, 271-286. DOI: 10.1007/s10540-005-2889-2.
The Encyclopedia of the Environment by the Association des Encyclopédies de l'Environnement et de l'Énergie (www.a3e.fr), contractually linked to the University of Grenoble Alpes and Grenoble INP, and sponsored by the French Academy of Sciences.
To cite this article: DIZENGREMEL Pierre (June 23, 2023), Plant resistance to stress: role of respiration?, Encyclopedia of the Environment, Accessed December 30, 2024 [online ISSN 2555-0950] url : https://www.encyclopedie-environnement.org/en/life/plant-resistance-stress-role-respiration/.
The articles in the Encyclopedia of the Environment are made available under the terms of the Creative Commons BY-NC-SA license, which authorizes reproduction subject to: citing the source, not making commercial use of them, sharing identical initial conditions, reproducing at each reuse or distribution the mention of this Creative Commons BY-NC-SA license.