Interaction radiation matter
PDF(See also the focus Overview of the physics of the atmospheric greenhouse effect)
1. Absorption, emission
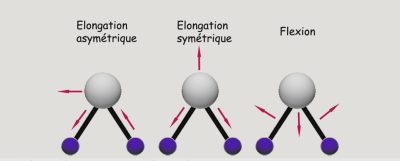
- the molecule as a whole moves at a speed directly related to temperature, the corresponding energy being kinetic;
- the electrons move around the nuclei in privileged orbits, so the energy is electronic;
- the atoms that make up the molecule are in motion relative to one another: the molecule is said to vibrate, and the energy is vibrational (Figure 1);
- the molecule turns on itself, and the energy is rotational (Figure 2).
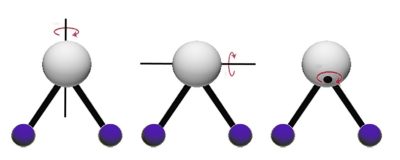
2. Selective absorption, excitation
A photon is absorbed when its energy where
is the frequency of the associated wave, enables a molecule to switch from one mode to another. The molecule being in the energy state
this occurs when there exists for it a state of energy
such that
. The molecule is then in an excited state, which it never remains for very long. The order of magnitude of the lifetime of excited vibrational states is close to
3. De-excitation
A molecule can de-excite in one of two ways: either by spontaneously emitting a photon of the same energy, known as radiative de-excitation, or by moving into the vicinity of another molecule, at such a distance that their electric dipoles interact. In the latter case, the additional energy is transformed into kinetic energy, known as a collision. The opposite process transforms kinetic energy into radiative energy when interactions between two nearby molecules trigger a transition to a more energetic state, with the molecule being excited by collision. After a very short time, it is in turn de-excited by one of the two processes, and so on. Absorption of radiation increases average kinetic energy and therefore temperature, while emission leads to the opposite phenomenon.
4. Excited state lifetime
The time the molecule remains in the excited state is called the excited state lifetime. In the case of radiative de-excitation, i.e. spontaneous emission, this is also called the natural lifetime. It can then be determined from the absorption coefficient or the Einstein coefficient [1] of spontaneous emission. For collisions, the lifetime depends on the density of the molecules and their agitation, i.e. pressure and temperature.
5. Hierarchy of transitions
Transitions between electronic levels are the most energetic, with the frequency
the frequency of the transition is in the UV or visible range, transitions between vibrational states
are located in the near IR, transitions from one rotational state to another
are the least energetic, in the IR and microwave ranges. In the event of a collision, a molecule can undergo all three types of transition at the same time, as well as if it interacts with a photon of frequency
such that
is a combination of
6. Local thermodynamic equilibrium (LTE)
For a gas in a closed enclosure, at constant temperature, which defines strict thermodynamic equilibrium, the various possible energy levels are populated according to Boltzmann’s statistical law [2], emission follows Planck’s law (see focus on Planck’s theory) and the radiation field is uniform. In the atmosphere, temperature is not constant, but molecular agitation ensures that energy levels are populated according to Boltzmann’s law [3] up to high altitudes, which vary according to the transitions, but are typically of the order of 40 to 50 km. In this case, known as Local Thermodynamic Equilibrium (LTE), the radiation field differs from that of the black body (i.e., the radiation is not uniform), but the molecules emit according to Planck’s law.
To challenge the ETL, we need to look at absorption or emission by the highest layers of the atmosphere (in practice, in the mesosphere, above 50 km for certain gas transitions, and generally much higher). The effect of ETL deviations can be seen when examining absorption spectra measured from satellites when observing at the limb, i.e. tangentially to the atmosphere, enabling us to probe its outermost layers, as with the MIPAS instrument [4] on the ENVISAT satellite [5].
Notes and references
Focus thumbnail. Extract from figure 2 of the focus, © figure by the author.
[1] https://fr.wikipedia.org/wiki/Coefficients_d%27Einstein
[2] Bear in mind that the number of molecules to be considered is very high. Typically, at standard conditions of pressure and temperature, there are 6.02 1023 molecules per 22.4 l (Avogadro’s number) or 2.68 1019 molecules per cm3. For carbon dioxide, that’s another 1.1016 molecules per cm3. All these molecules are distributed over the various possible energy levels, and it’s the statistical distribution of this distribution that depends on temperature via Boltzmann’s law.
[3] https://fr.wikipedia.org/wiki/Statistique_de_Maxwell-Boltzmann
[4] https://earth.esa.int/eogateway/instruments/mipas/description