The origin of pigment colours
PDFFlorent FIGON, Associate Professor, LECA/Inspé de Grenoble, Université Grenoble Alpes
1. When the colour diversity of butterflies is explained at the nanoscopic scale of electrons and photons
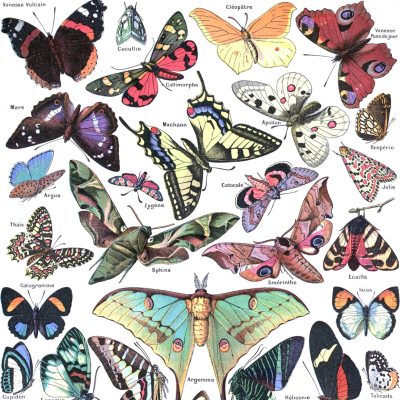
Yet, the colours are ubiquitous in the world as we perceive it (as evidenced by the beautiful colours of the butterflies drawn in the Figure 1), which raises the question of how some molecules are able to absorb at higher (and therefore less energetic) wavelengths than average.
These molecules, with their intriguing absorption properties, are called pigments [1]. To understand where their originality comes from, we need to explain the phenomenon of interaction of matter with light in wavelengths between 200 and 1,000 nm (from ultraviolet to near-infrared, including visible).
2. The interplay of photons and electrons
Light is made up of particles called photons, which possess a certain energy (which also defines the wavelength, which is inversely proportional to it). Matter is made up of molecules, themselves the result of interaction between atoms, themselves composed of a nucleus and electrons. It is these negatively-charged particles that are capable of interacting with photons in the wavelength range of interest.
This is because electrons have their own energy at rest, and are capable of moving to a small number of higher energy levels if, and only if, they absorb the amount of energy exactly equal to the difference between the energy of their initial and final states. It’s clear, then, that not every photon can excite every electron: an electron can only absorb a photon if the latter provides not only sufficient, but also exactly the right amount of energy to move to a higher energy state.
To make an analogy, electrons function like radio antennas, whose properties only allow them to listen to certain frequencies, since only a small fraction of them interact with the antenna. In the case of a DNA molecule, its electrons can only be excited by energies corresponding to photons located in the ultraviolet. Any other photon further away in the light spectrum, particularly in the visible range, passes through the DNA molecule as if nothing had happened.
It’s clear, then, that for a molecule to be coloured, it must have electrons capable of either starting at higher-than-average energy levels, or moving to lower-than-average energy levels, since photons in the visible range carry less energy than those in the ultraviolet. However, nothing is more like an electron than another electron, and so, taken in isolation, the electrons in pigments are the same as those in DNA. In fact, it’s their chemical environment that modifies their energy levels.
3. Pigments, molecules unlike any others
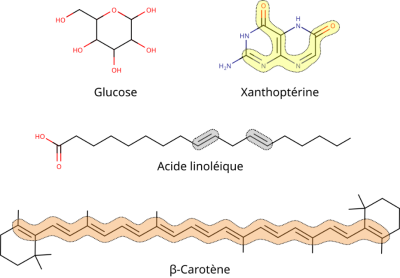
When pigment structures are compared with those of other transparent organic molecules (Figure 2), it becomes clear that a number of configurations between atoms are responsible for changes in their electron properties (it’s important to note that the following parameters are neither necessary, exclusive, exhaustive nor even sufficient:
- a sufficiently extensive alternation of single and double bonds, known as conjugate bonds;
- the presence of aromatic rings with conjugated bonds;
- the presence and positioning of heteroatoms (nitrogen, oxygen, sulphur, etc.) directly linked to conjugate bonds/aromatic rings;
- interaction with other compounds such as proteins, metals, etc.
4. The electronic origin of pigment colour diversity
The same properties that enable pigments to be coloured are also responsible for their different hues. Indeed, any variation in one of the above parameters can lead to the electrons in a pigment absorbing at different wavelengths. The colour we perceive in a pigment is the complementary colour of the one absorbed, which means that a violet-absorbing xanthopterin (yellow bands on Wasps) appears different from a blue-absorbing erythropterin (orange spot on an Aurora; Figure 3).
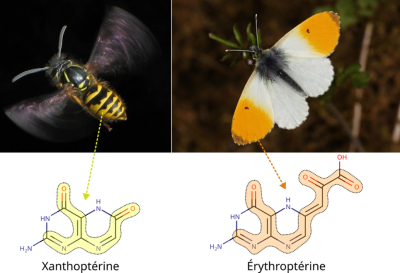
So there’s no clear dividing line between what’s visible and what’s invisible, only a gradient of absorption in the light spectrum according to the characteristics of the organic molecules (in this respect, pigments are nothing really special compared to all other organic molecules, apart from delighting our eyes).
In fact, when we look at the wavelengths actually absorbed by a pigment (using the UV/visible/IR spectroscopy technique that produces absorption spectra; Figure 4), we see that pigments don’t absorb at a single wavelength, but often at several points in the spectrum and over continuous ranges, forming more or less broad peaks around a mean value (Figure 4). This is because pigment electrons are influenced by the complexity of their environment (solvent, matrix, protein, etc.), enabling them to absorb a whole range of wavelengths relatively close to one another.
Ultimately, the energy levels of a pigment’s electrons dictate its total absorption spectrum in the UV-Visible-IR, which, together with other colouring mechanisms not discussed here, is what gives an organism its visible colour (Figure 4).

Learn more
- Figon, F. & Lorin, T. (2019) La coloration des Animaux : éléments de physique et de chimie. Planet-Vie. https://planet-vie.ens.fr/thematiques/cellules-et-molecules/biophysique/la-coloration-des-animaux-elements-de-physique-et-de (in French)
- Valeur, B. & Bardez., E. (2015) La lumière et la vie, une subtile alchimie. Paris : Belin. (In French)
Notes and references
Vignette : Danaus plexippus [Muséum de Toulouse, Licence CC BY-SA 4.0, via Wikimedia Commons]
[1] From a biochemical point of view, pigments can come directly from food (e.g., green bilines derived from plant chlorophyll, plant flavonoids kept intact; see Leaf colours) or from the body’s own metabolism (e.g., melanins, pterins and ommochromes/papiliochromes are produced from amino acids or nucleic bases, respectively tyrosine, guanine and tryptophan).