磁层:在地球与太阳的作用之下
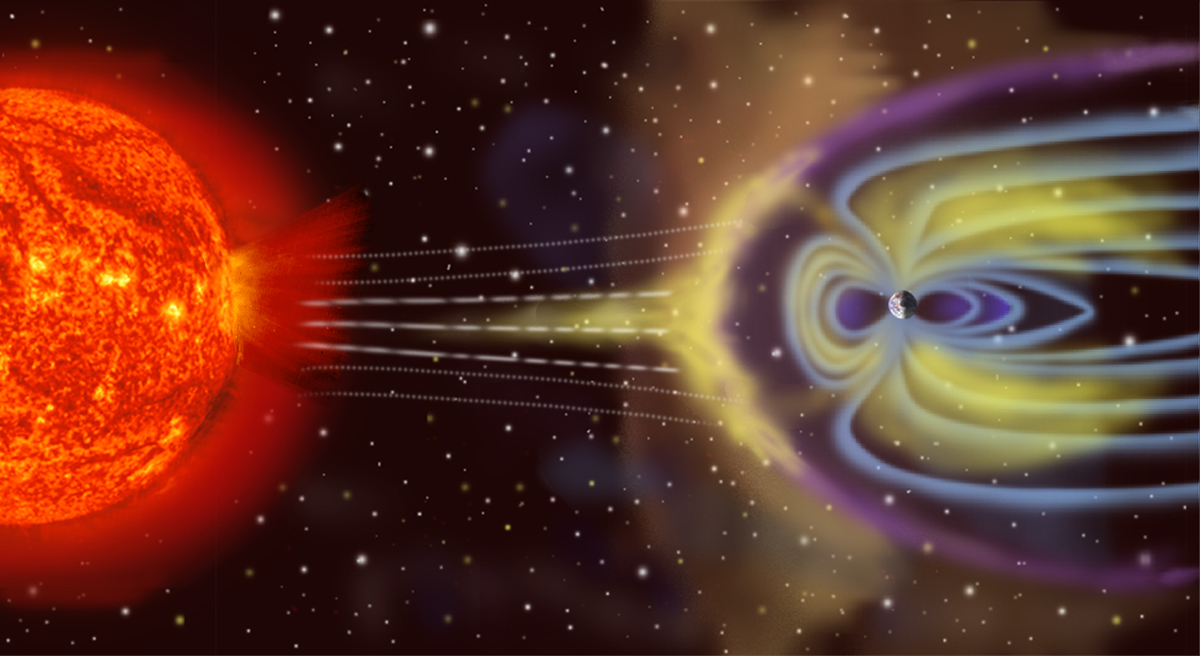
磁层是行星际环境和地球上空大气层之间的分界层,是在地球磁场作用下不断演化的广阔区域。磁层充满了无碰撞的等离子体,热力学持续不平衡。磁层内部动荡不定,几乎每天都在整体重构。磁层唯一的视觉呈现极具美感:那就是极光。
1. 太阳风
太阳的能量多以光的形式来到地球,包括红外光、可见光、紫外线以及含量较少且占比多变的X光。太阳还会将其中的极少部分以十分分散的物质风形式射到地球,组成成分有自由电子、质子和少量氦原子核。这些成分带有电荷,称为等离子风,或简单称作太阳风。太阳风的密度极低,每立方厘米一般仅有10个粒子,但速度很快,在地球轨道上每秒可达到300至800公里。也就是说,从太阳抵达地球轨道只用三天时间。太阳风带来的除了离子体,还有日冕的磁场。据估计,太阳风给地球环境带来的能量约为100GW(吉瓦)。比较而言,这些能量比法国所有发电厂生产的总发电量还要略高。当然,与地球每秒接受的太阳总能量(1017瓦)相比,这并不多,但足以在地球环境中产生显著的电磁效应。
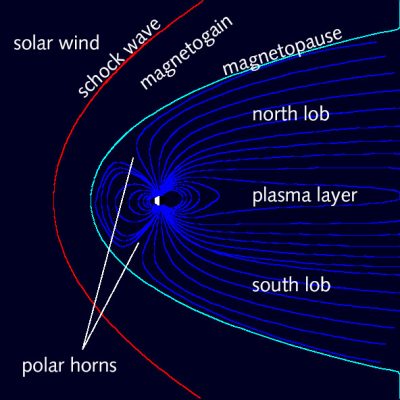
(图中solar wind:太阳风;shock wave:冲击波;magnetogain:磁鞘;magnetopause:磁层顶;north lob:北瓣;plasma layer:等离子体层;south lob:南瓣)
太阳风到达像水星或月球那样没有大气层的行星时,会直接作用于星球表面,特别是会非常缓慢地侵蚀土壤。太阳风到达金星或火星这样的行星,侵蚀的是上层大气,还会带走星球的一些成分。太阳风到达地球,情况则会更加复杂,因为地球本身的磁场会对太阳风的流动产生明显影响。反过来,太阳风也会改变地球环境的磁场和等离子体,在太阳风穿过的行星际环境和地球上空的大气层之间形成了一个分界层。分界层自太空时代之始的1957年得以探索,1959年获名:磁层。
2. 磁层
这一名称并不意味着磁层区域有着球体的形状,只是说明分界层处于地球磁场的作用之下,并且完全消失于行星际空间。图一显示了地球磁层的横截面:图中,地球是中心的小白点,太阳风来自左侧,图中深蓝色的线是相应的磁场,浅蓝色的线是磁层顶,红色的线是我们之后会谈到的冲击波。
磁层是动态的,似乎永远不会达到平衡。磁层不具稳定性,由于太阳风推力和磁力的持续变化以及一系列内部过程的影响,磁层几乎每日都会经历突然的重构。
探讨地球磁层的路径有两种。第一种旨在描述磁层(科学家实现的目标)并了解支配它的物理机制。第二种与第一种互补,通过预测磁层活动警示经济部门(工业、电网、运输)磁层所引起的电磁环境的波动。本文重点介绍第一种;第二种在《太空天气及其对地球的影响》一文中阐述。
磁层内部边界是电离层,离地球最近,是高空大气层(距地面60公里),这里中性气体与电离原子相互混合,出现等离子体。与中性大气层中的原子不同,这些带电的原子致使电离层成为良好的电导体。在电离层下部,即高度400公里以下,中性气体和等离子体仍相对密集,这里的粒子(原子、分子、离子和电子)彼此碰撞较为频繁。碰撞引起粒子之间脉冲和能量的交换,进而促进了整个电离层的局部热力学平衡的快速建立。根据基本的热力学量:包括密度、速度、温度、压力以及电量比如电荷密度和电流等,我们可以描述全部的物理性质。
磁层中等离子体的密度很低,而源自太阳等离子体的能量很高。这种混合结构有着特别的属性:即粒子之间的碰撞极为罕见。一个粒子必须穿越十万公里才可与另一粒子碰撞。从科学角度讲,无碰撞发生的平均行程距离约为100,000公里。由于这也是磁层的特征维数,即可推出磁层的等离子体是由彼此不会碰撞的粒子构成,称作无碰撞的等离子体。
无碰撞等离子体并不会在地球上自然产生,它们无法处于局部热力学的平衡之中(见《热力学》一文)。例如,来自电离层的温度低的等离子体与来自太阳的温度高的等离子体混合时,并不会得到温暖的等离子体,因为粒子的热搅动率并非是电离层的热搅动率和太阳风的热搅动率综合后的平均值。相反,运动范围小的粒子(温度低的那些粒子)和那些向各个方向运动的粒子(温度高的那些粒子)仍然处在相同的位置。的确,从数学角度来看,温度总是可以定义的,但是却不足以给出磁层等离子体的精确状态。对于习惯于日常和实验室环境中流体的物理学家而言,这种现象完全不和常理、令人困惑。
等离子体间的无碰撞造成了磁层的特殊属性,令磁层不具粘性,容易产生湍流。粘度的概念可以这样理解,如果把也太蜂蜜放入管中,蜂蜜在管中的流动非常缓慢,边缘的蜂蜜自身几乎不动,还会减缓蜂蜜的流动。我们把蜂蜜换成粘度低得多的水做同样的实验,流动无疑更快,但仍不均匀。由于粘度的作用,管道中间的水会比靠近管壁的水流动速度更快(水流的状态可以通过观察河流的流动更好得以理解)。由于粘度较低,水比蜂蜜更具湍流运动形态。而磁层的无碰撞等离子体几乎无粘性,湍流效应则更加放大。这令它无法达到平衡状态,即便最轻微的平衡状态或是局部而短暂的平衡状态也无法达成。
最后,就像任何等离子体一样,磁层的等离子体也由电粒子组成,而电粒子的运动受电磁场特别是地球磁场的控制。反过来,由于带电粒子在运动,也会产生电磁场。因此,这种等离子体改变着地球的电磁环境。
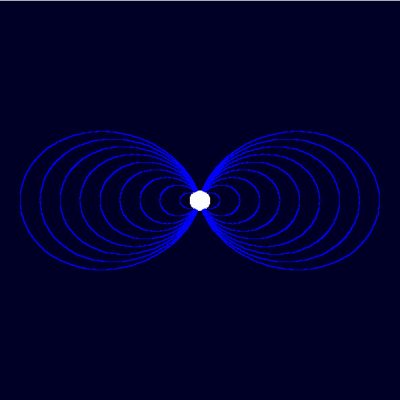
如若不存在磁层和太阳风,地球周围就会形成偶极磁场(如图2所示)。它会在空间中无限延伸,并随着距离变远而降低强度。由于太阳风的作用,磁场线呈现出图1所示的样子。图中可见:磁层具有非常细长的形状,在太阳一侧(白昼侧)从地球延伸出60,000公里,而另一侧(黑夜侧)则延伸出数十万公里。根据场线的地形,磁层可以划分几个区域,在空间探测上显示出不同的特性。
3. 磁层区域
磁层的最外层区域是冲击波,太阳风通过这里,速度从超音速降到亚音速。冲击波之后是磁鞘,一个充满湍流的等离子体区域,这一区域“噪声”较多,因为各种各样的极低频电磁波穿过此处。(不过人耳无法听到。)再之后是磁层真正的外边界:磁层顶。磁鞘中流动的仍是太阳风中的物质,磁场来源于太阳。而在磁层顶后,我们发现部分等离子体来自太阳,部分来自地球,且二者特征非常不同。只有一小部分太阳风能够通过磁层顶进入磁层。平均而言,磁层的物质密度低于太阳风的物质密度,结构就像形状复杂的泡沫,它的磁场源于地球。沿着磁场线在一个方向上运动,最终总会到达地球的电离层。磁层的最为复杂的区域在黑夜侧,分为向南向北两瓣(见图1),其中几乎没有等离子体。
4. 磁层活动
两瓣之间,磁场较弱的区域称作电流层,该区域的磁场可改变方向。磁层的大部分等离子体聚集于此。来自太阳风的等离子体到达磁层时,电流层会被填满,并且从东到西的电流不断穿过该区域(垂直于图1中的平面)。这也是最不稳定的区域:当等离子体积累偏多时,电流层会变薄(看似很矛盾),而电流会增强。最终,整个区域就会瓦解。
电流层如何瓦解呢?研究人员已经发现了几种机制:“磁重联”、“交换不稳定性”,但这仍是一个广受关注且极具争议的研究课题。
究竟发生了什么?电流层在几十分钟内清空。一些等离子体进入太空,另一些进入地球。这些电流层突然清空的重构事件称为磁层亚暴。一般来说,每天都会发生一个或多个强度不同的磁层亚暴或伪触发,释放出或多或少的等离子体和或强或弱的能量。表现为可见和壮观的极光,我们将在第6部分讨论。
5. 磁层中等离子体的加速
研究磁层时,我们将电子伏特(eV,读作能量)作为衡量粒子能量的单位。太阳风的粒子通常以100电子伏特能量进入磁层,等同于受到100伏的电压加速。电离层中的粒子具有0.1电子伏特的能量,但逃逸到磁层中的粒子通常会获得几电子伏特的能量。磁层尾部的粒子能量变化较大,从10电子伏特到50千电子伏特。通常,粒子能量的量级为100电子伏特。在亚风暴期间,能量会增加数百电子伏特。加速机制多有不同,与波、磁场和相应电场的变化相关。
6. 极光
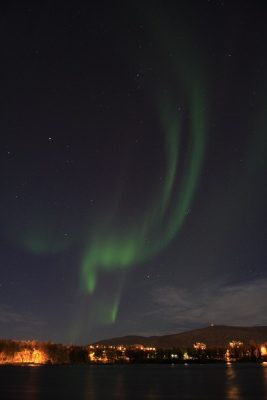
等离子体经过重构后,在几千公里的高度朝向地球实现另一轮加速。这是由磁层尾部重构过程中产生的电磁波和电结构引起的。加速最多的粒子是来自等离子体层的电子,能量可达几千电子伏特。当粒子到达电离层(电离层比磁层密度稍高并且存在粒子间碰撞)时,会在失去动能和速度之前挤掉几十个原子和分子。那些原子因为碰撞被“激发”,又因为发射光子“重回原态”。光子的能量对应于发生碰撞的原子的特性。例如,极光的典型绿色(图3)来自氧原子的碰撞。其他产生的颜色还包括:红色、紫色、蓝色。原子碰撞产生的光是磁层唯一可见的表现形式,多么辉煌的表现形式:一派壮观的景象,那就是极光。
最为明亮的极光都与磁层等离子体层的重构有关,所以全部发生在亚暴期间。在亚暴发生前的短暂时间里,虽然也可以观察到极光,但它们不那么明亮并且呈现出十分平静的形态。电流层的填充期通常在这时刚刚结束,“泄漏”开始发生。
太阳风就像风那样也会呈现风暴,每年都会发生几十次,但是强度不同。这些风暴不仅会影响太阳风的密度和速度,还会引起磁场强度以及方向的变化。有时风暴还会压缩地球的磁层。地球的磁层一旦受到压缩就会进入弛豫阶段,磁层的活动在两三天内会比平常更加强烈。亚暴因此更加剧烈、更加频繁,导致极光出现在并不常见的地方(例如在法国)。风暴形成磁暴。空间天气(参见《太空天气及其对地球的影响》)的目的即是预测那些可能对人类活动产生重大影响的磁暴。
环境百科全书由环境和能源百科全书协会出版 (www.a3e.fr),该协会与格勒诺布尔阿尔卑斯大学和格勒诺布尔INP有合同关系,并由法国科学院赞助。
引用这篇文章: MOTTEZ Fabrice (2024年3月11日), 磁层:在地球与太阳的作用之下, 环境百科全书,咨询于 2025年4月15日 [在线ISSN 2555-0950]网址: https://www.encyclopedie-environnement.org/zh/air-zh/magnetosphere-under-influence-earth-and-sun/.
环境百科全书中的文章是根据知识共享BY-NC-SA许可条款提供的,该许可授权复制的条件是:引用来源,不作商业使用,共享相同的初始条件,并且在每次重复使用或分发时复制知识共享BY-NC-SA许可声明。