Geothermal energy: a significant source of energy?
PDF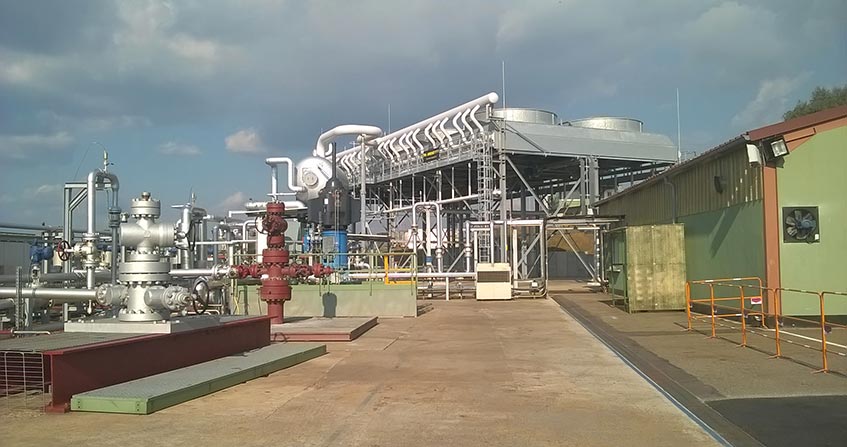
The heat contained in aquifers for which the temperature is between 50 and 120°C can be used for district heating provided that the point of heat production is fairly close to the point of consumption (about ten kilometres). When the aquifer temperature exceeds 200°C, this heat can be converted into electricity, allowing operation at much greater distances from the point of production. The French reserves allowing such exploitation are currently limited to natural aquifers only. But recent research results suggest that the heat from hot, low-permeability rocks may be exploited. The contribution of geothermal energy exploitation to national energy production could then become quite significant.
1. Heat and its transfers within our planet
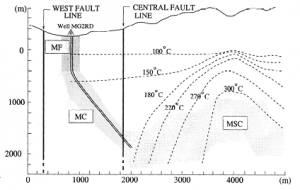
The notion of temperature is linked to that of heat, a form of energy whose transfers affect the temperature locally (read Pressure, temperature and heat). A distinction is made between heat transfers through conduction, heat transfers through convection, and heat transfers through radiation”.
Radiation transfers are associated with photon movements and are only significant on the surface of the globe. They are negligible for understanding temperature variations within the Earth. Conductive transfers, described by Fourier’s law [1], occur when a hot rock is in contact with a cold rock. Some of the heat contained in the hot rock is transmitted to the cold rock by conduction through the interface that separates the two bodies. In addition, rocks are more or less porous and more or less permeable and the pore volume generally contains a liquid that moves and thus transports heat.
An example of heat transfers involving both conduction and convection transfers is provided by a volcanic eruption (see The issues of industrial hydraulic fracturing). The heat transport associated with lava flow corresponds to a convection transfer: the volcanic eruption evacuates from the magma chamber a quantity of heat proportional to the mass of lava emitted during the eruption. Some of this heat is transmitted to the walls of volcanic pipes and is diffused in their vicinity, in the rocky massif, by conduction.
Two concepts related to these heat transfers are particularly important: heat capacity and thermal conductivity. The first is to estimate the amount of heat associated with a certain mass of material. The second describes how heat is transferred from a warm volume to a colder volume without material movement. For example, in a fluid at rest there is heat transfer from the hottest to the coldest areas due to the thermal conductivity of the fluid alone. However, the density and viscosity of a fluid depends on its temperature. As a result, temperature variations can generate convection movements whose velocity depends on viscosity, which in turn depends on temperature.
Thus, to fully understand temperature variations with depth, it is necessary to take an interest in the various fluid movements and to identify the sources of heat present inside our planet. The focus associated with this article introduces some fundamental notions about the structure of the globe. In particular, the definition of the lithosphere with solid mechanical behaviour and that of the asthenosphere with fluid mechanical behaviour are recalled.
A large part of the heat sources is related to the natural radioactivity of elements in the earth’s crust. In addition, a significant source of heat comes from the initial formation of the Earth and is associated with the nucleus. As a result, the temperature at the base of the lithosphere depends on the heat transfers associated with the convection movements that affect the lower mantle, i.e. the asthenosphere.
In the deep part of the earth’s crust, the geothermal gradient depends essentially on three elements. It depends first of all on the heat flux from the asthenosphere, i.e. a quantity of heat per unit area (expressed in watts per square metre in the International System of Units). It then depends on the thermal conductivity and the amount of heat produced by the natural radioactivity of elements in the upper mantle. Finally, it depends on the thermal conductivity as well as the local natural radioactivity in the crust. For the most superficial parts of the crust, the presence of fluids in the porous space of the rocks can involve significant convection movements. These then directly affect the regional temperature field. It is such convection zones that can be the site of very high temperatures at shallow depths (e.g. 300°C at 900 m depth on the island of Leyte in the Philippines, see Figure 1). It should be noted that when the pressure in the fluid is sufficiently low, it can exist simultaneously in its liquid phase and in its gaseous phase (e.g. presence of water vapour bubbles in liquid water). In addition to the mass heat already mentioned, latent heat associated with changes in the liquid-vapour phase transition must then be taken into account.
2. Temperature variations near the ground surface
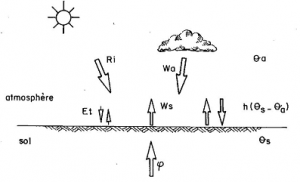
These mechanisms are as follows:
- evapotranspiration (term Et),
- the absorbed solar radiation (term Ri),
- atmospheric radiation (term Wa),
- the radiation of the ground (term Ws),
- a convective exchange term (h[θs-θa]) between the ground at θs temperature and the atmosphere at θa temperature,
- heat flux withf deep origin.
In prectice, observations have shown that the heat flux of deep origin is generally negligible as compared to the other sources of heat, except on volcanoes. Therefore, the surface ground temperature differs little (a few degrees) from the air temperature. Variations in solar radiation impose temperature variations on the surface of the ground, related to daylight or seasonal variations.
These temperature variations are propagated in the underlying soils, in particular due to their thermal conduction. Hence, because of conduction alone, diurnal variations affect the first 15 cm of soil. The annual variations imply temperature variations over the first three metres, while secular variations can reach the first 30 metres. At a scale of 10,000 years, variations affect soil temperature by conduction to a depth of 300 m. Thus, the trace of the last glaciation (apogee about 20,000 years ago) can be found in thermal profiles (variations in the geothermal gradient with depth) up to about 400 to 500 metres deep, depending on the effect of disturbances related to fluid circulation.
It is remarkable that temperature variations over periods of less than a year are only in the order of a few tenths of a degree as soon as one reaches a few metres below the surface of the ground. For example, annual measurements, repeated over a few years and carried out in a granite outcropping at an altitude of about 500 m in the Vichy region, revealed seasonal temperature variations of just one-tenth of a degree at a depth of 15 m below the ground surface. It is this stability of the shallow temperature that allows the installation of so-called geothermal heat pumps, as discussed below.
3. Conventional methods of exploiting geothermal energy
An aquifer is a highly permeable geological level with high porosity and pores filled with water. It should be remembered that permeability characterizes the ease with which fluids flow through rocks. It relates the flow rate observed between two given sections to the pressure difference between them. For the same flow rate, the more permeable the rock, the smaller the pressure difference required to ensure this flow rate.
Conventional geothermal energy exploitation only concerns natural aquifers and consists in extracting the heat contained in the aquifer by bringing its water to the surface of the ground at a temperature close to that of the aquifer.
There are traditionally three types of geothermal energy exploitation: exploitation by heat pump, direct exploitation of the heat contained in aquifers, and finally, when the temperature allows it, electricity production after conversion of heat from geothermal sources.
In France, for the year 2012, the energy production associated with geothermal heat pumps installed for individual heating was equivalent to just under 300 ktoe (kilotonnes of oil equivalent), or about 3.4 GWh (Giga Watt hour) [4]. The energy production linked to the direct exploitation of geothermal heat amounted to 140 ktoe (about 1.6 GWh) for the same year 2012. In 2016, the geothermal electrical power installed in France amounted to 17 MW (Megawatt), representing an annual production of approximately 149 GWh if we assume full-time operation of the installations over that year. To better appreciate the current contribution of geothermal energy exploitation to national energy production, it should be recalled that the quantity of electrical energy produced in 2013 by French nuclear power plants alone amounted to 391,000 GWh.
3.1. Heat pump operation
A heat pump is a device for transferring a certain amount of heat from a first medium, called an emitter, to another medium, called a receiver. The system thus makes it possible to lower the temperature of the emitting medium and increase the temperature of the receiving medium.
For example, a heat pump is used to lower the temperature in a refrigerator (emitting medium), but it can also be used to heat a room (receiving medium). Moreover, the same heat pump principle can be used to cool a room if it is used as a emitting medium, and no longer as a receiving medium. In the case of the refrigerator, heat extraction is carried out by the expansion of a fluid, known as refrigeration, which changes from liquid to vapour by pumping the heat into the refrigerator, resulting in a decrease in the local temperature. This vapour is then compressed back to the liquid phase outside the refrigerator where the operation emits heat, raising the local ambient temperature.
The coefficient of performance (COP) of a heat pump (PAC) is defined by the ratio between the thermal power of the machine and the electrical power consumed. For example, a heat pump with a COP of 3 provides 3 kWh (kiloWatt hour) of heat for every 1 kWh of electricity consumed. The coefficient of performance of a heat pump decreases as the difference between the temperatures at the cold and hot source increases. Thus, if the temperature of the cold source is constant, the efficiency of the heat pump decreases with the temperature of the hot source. As a result, so-called geothermal heat pumps, i.e. heat pumps with a cold source located in the basement, are more efficient when used to ensure a constant temperature in a floor, for example. In this case they provide a minimum basic heating, the complement being provided, depending on the needs, by electric convectors.
Geothermal heat pumps use three types of heat capture in the basement:
- Horizontal capture. The heat is captured by a network of parallel horizontal tubes through which a heat transfer fluid flows. These tubes are buried between 60 cm and 1.2 m deep, depending on the climate. They are placed under a lawn, but away from trees whose roots could disturb the installation.
- Vertical capture. Vertical capture involves a series of parallel tubes distributed in a vertical borehole that can reach a depth of up to 100 metres. This borehole, itself being solidly tubed (read Some characteristics of drilling techniques), can be placed near trees.
- Vertical collection on groundwater. In this case, the water from the local groundwater is used directly as a heat transfer fluid. It is pumped through a so-called suction borehole. A groundwater temperature of 10°C or more is targeted. The water from the groundwater circulates in the heat pump emitting enclosure located in the house. It is discharged into a borehole downstream of the suction borehole so as not to disturb the temperature.
3.2. Direct use of heat
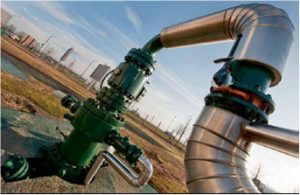
Reinjection is necessary for two reasons. The first is that water in aquifers of economic interest (more than 500 m deep) is very generally loaded with various mineral salts (at concentrations of 6 to 35 g/l or more). It should therefore be purified before its release if it were to occur in the hydrographic system at the surface of the ground. In addition, the reinjection ensures that the tank is maintained under pressure, which ensures a constant flow rate during production.
Geothermal energy is therefore not strictly speaking a renewable energy, because the amount of heat extracted from the geothermal reservoir is much greater than the amount of heat provided by the regional heat flow from the deepest depths.
The dipoles must therefore be properly dimensionned (production rate, sufficient distance between injector and producer wells) to avoid their premature cooling. Another dimensioning element has long been corrosion, which affects the various elements of the geothermal loop. But after having caused a problem when this type of operation started in the 1970s, this corrosion problem was well solved and no longer affects the service life of the doublets. Today, the oldest dipoles have not experienced premature cooling for most of them and are therefore still effective. However, their inevitable ageing requires that solutions be found for their renewal when the cooling becomes too significant. Current research is therefore focused on obtaining a good characterization of the extension of the areas cooled by the operation, in order to define the areas where future replacement doublets will be installed.
This type of exploitation has developed particularly well in the Paris Basin, where the Jurassic limestone geological layer of the Dogger has proved to be a very interesting aquifer. It has also been somewhat successful in the Aquitaine basin. But to be profitable, these geothermal operations must be located at short distances from heat use points (usually district heating) due to energy losses during the transport from the production well to the heating point. Their field of application thus remains essentially limited to urban areas in which there are enough consumers at an acceptable distance from the doublet, as for example in the Paris region.
3.3. Electricity production by converting heat into electrical energy
When the aquifer temperature is high enough, the heat produced can be efficiently converted into electricity through a converter located next to the producing well (see cover image). A geothermal reservoir is a volume of rock large enough to allow for the exploitation of its geothermal energy for a period of time of real economic interest. This typically reaches a few decades, even more so in Larderello in Italy where the field was first exploited in 1904. Today, such geothermal reservoirs for electricity production involve aquifers generally at temperatures above 250°C. Production can vary, depending on the reservoir, from a few tens of MW to several hundred MW, or even exceed the GW as in the Geysers reservoir in northern California.
The profitability of a geothermal reservoir depends, of course, on its temperature field, but also significantly on the volumes of water that can be produced in a stable way over time. The higher the temperature of the reservoir, the lower the flow rate required to be economically viable. In reservoirs that have been in operation for some time, there is a local drop in pressure that can lead to the appearance of a vapour phase in the produced water, which affects the ageing of the system. This notion of aging is one of the important factors to consider when determining the profitability of a geothermal operation.

4. Exploiting the heat from warm, low-permeability rocks

We note a strong heat flux anomaly in the northern Rhine graben, as well as a fairly high heat flux zone (over 110 mW/m2) over a large portion of the territory extending from the centre of the Massif Central to the Vosges.
For example, the temperature reaches 140°C at the base of the sedimentary series encountered at a depth of 1500 m in Soultz-sous-forêts, a village in the northern Rhine graben. It reaches 160°C at the basement-sediment boundary encountered at a depth of 2300m at Rittershoffen located about fifteen kilometres east of Soultz. However, while drilling in Rittershoffen has reached aquifers with satisfactory water production characteristics for direct economic exploitation of heat, the same has not been the case in Soultz. For this site, which is hot but too low in permeability for conventional operation, a new operating method called EGS (based on the initials of the English words Enhanced Geothermal Systems) has been developed to enable small electricity production (1.5 MW).
The EGS method is based on the idea that it is possible to considerably increase the permeability of a rock mass by appropriate hydraulic and chemical stimulation. The objective is to circulate, after stimulation, water flows compatible with the planned economic exploitation, and this at local flow rates slow enough so that the temperature of the water produced at the surface is close to that of the stimulated area. For this type of application, it is not possible to use the conventional hydraulic fracturing process (read The challenges of industrial hydraulic fracturing) because granular products, set up to keep a hydraulic fracture open at the end of the fracturing operation, tend to be dissolved by circulating water. It is also necessary that there are enough flow channels in the rock to avoid premature cooling of the system.
The method adopted at Soultz consists in gradually increasing the pressure in the deep rocky massif, in order to induce shear movements along the pre-existing fractures used by water flows. Similarly, acid injections have made it possible to dissolve calcite locally and thus reduce the hydraulic impedance of the system (the hydraulic impedance, inversely proportional to permeability, makes it possible to characterize the pressures required to reach given flow rates). In addition, the efficiency of the process of converting heat into electricity has been improved by using a liquid at a much lower boiling point than water. This experimental system has been operating satisfactorily in its current configuration since summer 2016. It will allow us to test, in real life, the ageing of the system.
Once these ageing studies are completed, the EGS process should make it possible to consider exploiting the huge geothermal reservoir that could be constituted by the heat flow zones above 110 mW/m2 shown in Figure 5. The contribution of geothermal energy exploitation to national energy production could then become quite significant.
References and notes
Cover photo. Electricity production in Soultz-sous-forêts (Bas-Rhin) from geothermal energy extracted at a depth of nearly 4500 m. In red, the head of the water-producing well at 150°C; in the background on the right of the wellhead, the binary converter producing 1.5 MW of electricity (photo, Électricité de Strasbourg)
[1] Berest P., 1988. Thermal phenomena in geotechnics; chapter 1 in La thermomécanique des roches (Berest and Weber ed.) presses of BRGM, also in Thermomécanique des roches (ed. P. Berest) to be published in 2018 by Presses de l’Ecole des Mines de Paris
[2] Prioul R., F.H. Cornet, C. Dorbath, L. Dorbath, M. Ogena and E. Ramos, 2000. An induced seismicity experiment across a creeping segment of the Philippine Fault; J. Geophys. Res. 105(B6), pp 13595-13612.
[3] G. Vasseur, 1988 . Propagation de la chaleur dans la terre et flux géothermique, chap. 4 in La thermomécanique des roches (Berest et Weber ed.) presses du BRGM, ; also in Thermomécanique des roches (ed. P. Berest) à paraître en 2018 aux Presses de l’Ecole des Mines de Paris.
[4] Boissavy C., P. Rocher, P. Laplaige and C. Brange, 2016, Geothermal Energy Use, Country Update for France, European Geothermal Congress.
[5] Lucazeau F. and G. Vasseur, 1989, Heat flow density data for France and surrounding margins, Tectonophyics, vol. 164 (2-4), pp 251-258.
The Encyclopedia of the Environment by the Association des Encyclopédies de l'Environnement et de l'Énergie (www.a3e.fr), contractually linked to the University of Grenoble Alpes and Grenoble INP, and sponsored by the French Academy of Sciences.
To cite this article: CORNET François Henri (January 5, 2025), Geothermal energy: a significant source of energy?, Encyclopedia of the Environment, Accessed April 6, 2025 [online ISSN 2555-0950] url : https://www.encyclopedie-environnement.org/en/soil/geothermal-energy-significant-source-energy/.
The articles in the Encyclopedia of the Environment are made available under the terms of the Creative Commons BY-NC-SA license, which authorizes reproduction subject to: citing the source, not making commercial use of them, sharing identical initial conditions, reproducing at each reuse or distribution the mention of this Creative Commons BY-NC-SA license.