光合作用的碳代谢途径
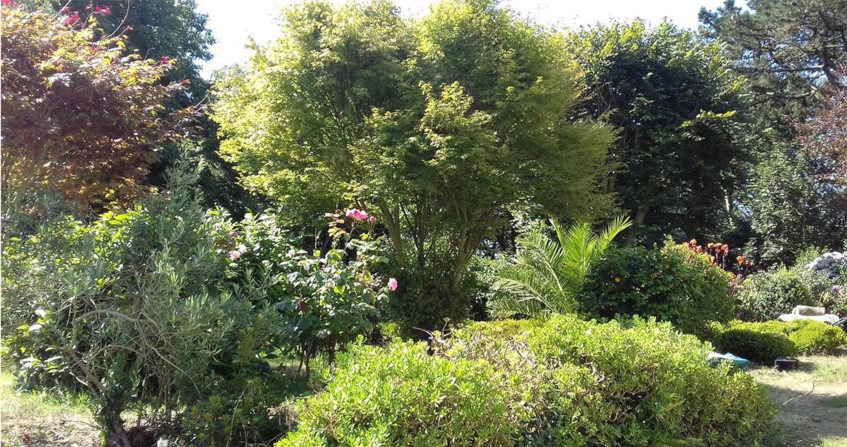
大气二氧化碳(CO2)中的碳是如何整合到有机物中进而构成生物体(生物量)的?数十亿年来,这一过程在生物光合作用的光化学阶段发生,利用叶绿素从阳光中回收能量来实现。然而,光合作用必须调整这些机制以适应地质时间尺度上的各种环境变化。光合作用光化学阶段产生氧气(O2)在大气中的富集(参阅“揭示光合作用的真相”)便是其中一个重要例证。在进化过程中,不同的独特策略生成了多样化的有机生物分子,用于人的衣、食、住、行。
1. 什么是光合作用
1.1. 利用空气中的二氧化碳来合成生物量

Simple metabolites(简单代谢物质):Sugar(糖),Starch(淀粉),Cellulose(纤维素);Fatty acids(脂肪酸),Lipids(脂质);Aminoacids(氨基酸),Proteins(蛋白质);H2O & Minerals(水和矿物质);Seconary metabolites(次生代谢物质):isoprenoids(类异戊二烯),Terpenes(萜烯),Carotenoids(类胡萝卜素),Sterols(甾醇),Chlorophylls(叶绿素);Phenylpropanoids(苯丙氨酸),Phenolis acids(酚酸),Flavonoides(黄酮),Liginins(木质素);Glycosides and Alkaloids(糖苷和生物碱)
光合作用是一种古老的机制(已有38亿年历史),是含叶绿素的绿色植物、藻类及光合细菌通过一系列生物物理和生化反应将阳光中的电磁能以及大气二氧化碳、水、土壤矿物质中的碳合成有机分子的过程(如图1)。
因此,光合生物是自养生物*。光合作用是生物食物链中大多数分子的起源,也是地球上大部分有机生物量的起源。光合作用在叶绿体中发生,这种数微米大小的绿色细胞器中蕴藏着光合作用的机制(见“揭示光合作用的真相”),光合作用的机制可简化为下式:
CO2 + H2O + 光能 → 能量-富含碳的分子 + O2
光合作用每年从大气二氧化碳中固定1150到1200亿吨碳,其中600亿吨来自大陆。而该过程仅用了照射到地球上太阳光能的很小一部分(约1-2%)。从全球规模看,其功率约为130-140兆瓦(1兆瓦=1012瓦)级别,相当于人类能耗的6倍。光合作用生物是怎样做到这些的呢?
1.2. 光合作用分为两个阶段
快速的光化学反应阶段,发生在叶绿体的膜系统——类囊体上(图1、2)。

Light(光),Photochemistry(光化学阶段),Photosystems ATPases(光合系统ATP酶),Biochemistry(生物化学阶段),Carbon metabolism(碳代谢),BBC Cycle (BBC循环),Sugars(糖类)
在此阶段,太阳光的可见光被叶绿体中的叶绿色素捕获。获取的能量随之传递给蛋白质/色素复合体(光系统),通过连续的氧化还原反应将光能转化为电能并进一步变为化学能,储存在富含能量的分子和还原态NADPH中。同时,类囊体膜两侧的质子梯度为生成ATP提供能量。在此过程中,水分子(H2O)是电子(e–)、质子(H+)和氧气(O2)的来源。这一过程在百科文章中有详细描述(见“揭示光合作用的真相”)。
- 代谢阶段,比前一阶段慢,发生在叶绿体内液-基质中(图2)。
大气二氧化碳中碳的固定及羧化反应进行需要酶作为碳受体方可发生并生成有机化合物。这一光合作用的碳代谢路径被称为本森-巴萨姆-卡尔文循环(Benson-Basham-Calvin Cycle)。
本文主要描述:
- 光合作用从大气二氧化碳进行碳固定的生化机制;
- 上述机制随环境变化的演化;
- 不同地质时期大气中氧气的出现及其影响。
2. 植物怎样从二氧化碳中固定碳
2.1. 一些历史
珍妮·瑟讷比埃(Jean Sénebier,参阅“研究光合作用的先驱”)早在1782年就首次描述“光合生物在光照条件下固定二氧化碳并维持植物生长”。从18世纪后期到20世纪40年代中期,通过何种路径从二氧化碳中吸收碳进行光合作用依然成谜。19世纪早期,布森戈(J.B Boussingault)和拜耳(F. Bayer)最初认为碳水化合物是由碳与水成分结合而成,由此糖得名为碳水化合物。大多数表示糖类的分子式实际可写成碳和水组成基本分子(CH2O)n的多聚化。之后,其他几个化合物也被认为是光合作用的初级产物,例如碳酸(H2CO3)、甲酸(HCOOH)等最简单的羧酸*。然而,那时没有实验结果能证实这些假设。甲酸是光合作用第一产物的假说之所以在很长一段时间内出现在文献中,也只是因为它极简单但能说明问题。
2.2. 本森-巴萨姆-卡尔文循环
- 对二氧化碳受体的寻找及特定羧酶的发现
图3. 本森-巴萨姆-卡尔文循环示意图。表示光合作用碳元素被引入及还原的步骤,包括形成3-磷酸甘油酸(PGA)、磷酸丙糖,光合作用第一中间产物,以及二氧化碳受体与核酮糖-1,5-二磷酸(RUPB)的再生。[来源:Schéma Roger Prat, in Morot-Gaudry, Dunod, 2009]
放射性同位素11CO2用作标志物进行的首个实验[1]显示,11C出现在一种三碳化合物中,表明二氧化碳的碳受体是二碳化合物。但这一假定的化合物一直未能确定。
直到14CO2用作放射性示踪剂后,本森[2]才发现14CO2中的碳元素结合入一种更复杂的原有碳结构:一个五碳磷酰基化合物,核酮糖-1,5-二磷酸(RuBP)(参阅“本森-巴萨姆-卡尔文循环的解密”)[3]。该化合物化学结构易于加碳(称为羧化反应),生成一个极不稳定的六碳化合物,并立即水解为两个三碳(C3)分子,即磷酸甘油酸(PGA[4])(图3)。
将二氧化碳的碳元素结合到RuBP上的酶是一个羧化酶*,即RuBP羧化酶,后文称RubisCO(见下文)。30亿年来,光合作用这一特定羧化酶是二氧化碳的碳元素进入地球上大多数有机分子的关键途径(参阅“RubisCO”)。
RubisCO是一种高分子量(550kDa)的复杂酶[5],存在于叶绿体基质中,占可溶性蛋白的30-50%。RubisCO是生物圈中最重要的定量酶,是叶片中有机氮的主要储备[6]。由于它在自噬中的核心作用,地球上每个人的生存都需要5千克RubisCO的合成。
- 磷酸甘油酸还原为磷酸丙糖
图4. 本森-巴萨姆-卡尔文循环中关于碳交换的简图。图中未显示磷酸化过程,只展示了分子中碳的数目。[来源:Schéma Roger Prat, in Morot-Gaudry, Dunod, 2009]
从光化学反应阶段还原态NADPH和ATP中恢获取能量(见“揭示光合作用的真相”),三碳PGA分子还原为(得电子)磷酸丙糖分子(含3个碳和一个磷酸分子)并获能。这一酶促还原中,每还原一个PGA分子,需要消耗一个ATP和一个NDPH。
- 磷酸丙糖的命运
每生成6个磷酸丙糖分子中,只有1个用于碳水化合物、氨基酸和脂质等的合成,其他5分子的磷酸丙糖将用于二氧化碳受体RuBP的再生(图4)。RuBP分子的再生耗能高,每分子需要消耗2分子NADPH和3分子ATP,不过这些能量是由太阳免费提供的。
由于这一循环的第一产物是一个三碳分子,具备该循环反应的植物被称为C3型光合植物。
其他未用于RuBP再生的磷酸丙糖将被用于以下方面:(a)在叶绿体中用于合成淀粉、氨基酸和脂质;(b)被转运出叶绿体,由细胞质中的酶转化为糖供进一步代谢(见“蔗糖或者淀粉?”)。
- 光合作用同化物的合成和运输
图5. 植物中原液和韧皮汁液的循环
[来源:©Jean-François Morot-Gaudry]。Photosynthesis (光合作用),Leaf(叶片),Sugar loading (载糖),Phloeme sap(韧皮部汁液),Xyleme sap(木质部汁液),Roots and tubers(根和块茎),Sugar unloading(卸糖)
植物传导系统中与运输原液的木质部平行的韧皮部能够运输大量汁液,将光合作用产物和同化物质运输并分散到植物各处(图5)。韧皮汁液的长距离运输要求在源器官树叶中合成的同化物(蔗糖,主要是氨基酸)通过一个主动和选择性的装载机制被装配到传导复合体中,之后被持续卸载到谷粒、种子、水果、块根和块茎等接收器官中。
2.3. 温度的影响?
温度对生物物理和代谢过程的影响不同。叶绿色素吸光及NADPH和ATP合成等生物物理过程对温度变化并不敏感。然而,二氧化碳和氧气固定和糖类合成、胞内和器官间分子交换等生化反应却有高度的温度依赖性。温度平均每升高10℃,生化反应的速率就会增加一倍。
在温带地区,空气和植物温度受强烈的季节性和日内变化影响,这些变化与到达地表的太阳能量变化一致。植物不同程度地适应了快速变化的日内早晚温差。叶片的温度通常会随日光变化而快速变化。当环境温度很低时,例如在阿尔卑斯山脉,植物形成了能应对这些温度变化的机制(见“植物如何应对高山胁迫?”)。
植物还可以适应长期的气候变化。在所有情况下,随着温度升高,光合作用随之增强。但对于一些植物来说,适应新的温度条件有可能会导致光合效率下降。
3. 不同地质时期的氧气产量
地球上第一次光合反应发生在三十亿年前,那时的大气层主要由水(H2O),二氧化碳(10%至15%),二氧化氮(N2)和硫化氢(H2S)组成,几乎没有氧气。那时,在将光能转换为含能分子的过程中,原始的光合细菌,如紫色亚硫酸细菌,会像绿色亚硫酸细菌一样氧化硫化氢,此时的光合作用不产生氧气。
随着蓝细菌祖先的出现,H2O变成取之不尽的氧化底物以及电子和质子的提供者,进而产生氧气释放到大气中,光合作用变成了产氧类型(见“揭示光合作用的真相”)。此时,这两种类型的光合作用共存:
CO2 + 2 H2S→(CH2O)+ H2O + 2S(无氧光合作用)
CO2 + 2 H2O→(CH2O)+ H2O + O2(有氧光合作用)
大约25亿年前,产氧光合作用出现之后,由于壳层矿物能够以氧化铁(Fe2O3)的形式大量捕获氧气,大气中的氧气浓度在很长一段时间内仍保持较低水平。地球历史中的这一阶段被清晰地标记在富含铁元素的红色地质层中(见“生物圈,重要的地质作用者”)。在所有矿物质都被氧饱和后,即在大约24亿年前的“大氧化”时期之后,由蓝细菌和真核生物的光合作用释放的氧气水平在大气中急剧增加。当浓度接近大气的21%时,氧给光合物种带来了严重问题。
4. 氧气,光合作用的灾难?
4.1. 更多历史
在20世纪20年代,奥托·瓦尔堡(Otto Warburg)[7]发现如果空气(含0.0408%二氧化碳)中的氧气含量从20%降低到2%[8],二氧化碳同化的净速率会从1.5倍增加到2倍,这被称为瓦尔堡效应:较高的氧气张力会抑制光照下二氧化碳的吸收。在20世纪70年代,鲍斯(Bowes),洛里默(Lorimer),奥格伦(Ogren)和托尔伯特(Tolbert)等通过18O氧同位素标记实验,证明了与二氧化碳结合的核酮糖二磷酸羧化酶也能够与氧结合[9]。
4.2. RubisCO的困境:氧气 / 二氧化碳竞争

因此,核酮糖二磷酸羧化酶除了发挥其羧化作用以外,还具有第二种作用,即加氧酶。所以RubisCO(核酮糖二磷酸羧化酶加氧酶)又被称为双功能酶(图6)。氧气和二氧化碳会在RubisCO的催化部位竞争,并参与同一分子的两种拮抗活性。
- 二氧化碳促进RubisCO的羧化酶功能;
- 氧气通过光呼吸过程促进加氧酶功能。
在高浓度二氧化碳存在的条件下,RubisCO仅起羧化酶的功能,合成两个PGA分子(C3),这两分子PGA是Benson-Basham-Calvin循环中磷酸糖的来源。
RuBP(C5分子)+ CO2(C1分子)→2PGA(C3分子)(羧化反应)
另一方面,当氧气浓度高、二氧化碳浓度低时,RubisCO起加氧酶作用,合成一个PGA(C3分子)和一个磷酸乙醇酸分子(或P-乙醇酸,C2分子)。
RuBP(C5分子)+ O2 →PGA(C3分子)+ 磷酸乙醇酸(C2分子)(氧化反应)
4.3. 磷酸乙醇酸循环

[来源:©Jean-François Morot-Gaudry]。Chloroplast (叶绿体),Peroxysome (过氧化物体),Mitochondria(线粒体),Glycolate(磷酸乙醇酸),Glycine(甘氨酸),Serine(丝氨酸),Glycerate(甘油酸)
光呼吸的第一产物2-磷酸乙醇酸已被证明是本森–巴萨姆–卡尔文循环的强大抑制剂。大多数植物通过复杂的2-磷酸乙醇酸循环来代谢去除这种有毒复合物(也被叫作氧化光合作用碳循环或Tolbert循环),该过程在叶绿体,过氧化物体*和线粒体*三种细胞器的协同作用下进行[10]。在此循环中,两分子的2-磷酸乙醇酸被转化为一分子PGA,然后重新进入本森-巴萨姆-卡尔文循环,同时,一分子CO2和一分子NH3被释放到大气中。
除了这些碳和氮的损失外,乙醇酸循环中也消耗了大量以NADPH和ATP形式存在的能量。但是,由于乙醇酸循环途径的开放性,光呼吸产生的大部分碳得以回收,从而限制了光合作用碳的损失(图7)。
光呼吸主要发生在一些生长在温带的C3型光合植物中(如小麦,大麦,番茄,生菜,土豆,树木)。据估计,在25°C的正常环境条件下,即21%的氧气和0.0408%的二氧化碳时,羧化和氧合速率之比约为2.5,即光合作用同化的二氧化碳有20%通过光呼吸排放。光呼吸的重要性与环境状况密切相关:
- 在温度和光照较强,二氧化碳浓度较低的情况下,光呼吸显得尤为重要;
- 相反,较高的二氧化碳浓度有利于羧化反应进行。
4.4. 光呼吸:主要的适应性过程
在超过30亿年的时间里,光合作用这一强大过程在适应地球重大环境变化的历程中一直非常稳定(见“生物圈,重要的地质作用者”)。光合作用代谢过程的演化与环境变化密切相关:
-
- 在大约7亿年前,随着环境中的氧气浓度增加,二氧化碳/氧气比率急剧下降,引起全球冰川作用。
- 在占领大陆之前,这些新的环境条件为微生物和藻类RubisCO发挥功能提供了氧压[11]。
- 为了适应这些新条件,绿色谱系(陆生植物的祖先)的分支发展出了光呼吸途径,从而在4亿3千万年前开始占领大陆。
- 一旦暴露在大气中,植物就必须应对新的进化压力,并且尝试不同的途径减少或避免光呼吸。
因此,光呼吸在光合过程不可或缺,因为它与RubisCO本身的固有特性有关,而这些特性是在氧含量几乎可以忽略的进化年代中形成的[12]。
5. RubisCO附近的二氧化碳富集
除了C3植物之外,其它一些光合生物(蓝细菌,C4和CAM植物……)也进化出了独特的策略,以有效降低氧气对RubisCO的有害效应。最显著的策略之一就是将二氧化碳浓缩在RubisCO酶附近。
5.1. 光合细菌:在RubisCO附近建立一个二氧化碳储藏库

[来源:©Jean-François Morot-Gaudry]。Carbonic anhydrase(碳酸酐酶),Carboxysome(羧酶体)
光合细菌的光合作用体系定位于其细胞膜上,因此,蓝细菌内形成了一些由多面体蛋白壳构成的微区室结构,即羧酶体,其中含有参与碳固定的酶(图8)。
这些结构使得蓝细菌可以生活在缺乏溶解性二氧化碳但富含HCO3–离子的水生环境中。其限功膜上特定的高效转运蛋白捕获碳酸氢根(HCO3–),并被碳酸酐酶*这样一个特定的酶转化为二氧化碳。这种机制使得RubisCO附近环境中形成了一个浓缩二氧化碳的胞内储存库,重现了古代地质时代原始大气环境。这降低了RubisCO的加氧酶活性进而提高羧化活性[13]。
5.2. 如何将二氧化碳固定与RubisCO物理性分离?C4植物的解决方案

图上内容:mesophyll 叶肉,Bundle sheath 鞘束, Cycle de CALVIN卡尔文循环
有些植物,例如玉米,也已经进化出富集二氧化碳的高效机制,在叶片内部,这一机制涉及到两种不同的组织(图9):
- 一种是围绕导管组织,位于最外围的叶肉细胞;
- 另一种是围绕最中心的组织,维管束鞘细胞(一种非常不透水的俄罗斯套娃状的结构)。
叶肉细胞中含有特定的羧化酶,磷酸烯醇式丙酮酸羧化酶或PEP-羧化酶,它们会催化合成四碳化合物-草酸乙酰(因此被称为光合作用或C4型植物)[14]:
PEP(C3分子)+碳酸氢盐(C1分子)→草酰乙酸(C4分子)
在叶肉细胞的叶绿体中,草酰乙酸被转化为另一种C4化合物苹果酸,并运往维管束鞘细胞。在这里,C4化合物经过酶促脱羧之后,形成大量CO2富集在RubisCO周围,从而促进其羧化活性。之后磷酸烯醇式丙酮酸再生,以确保持久循环。
这一机制可以将从大气中捕获CO2和在RubisCO中进行利用两个过程物理性地分开,但与C3植物相比也消耗了更多的ATP能量[15]。
5.3. 肉质植物中的时相分离:夜间进行C4代谢,白天进行C3代谢。
在肉质植物(仙人掌,凤梨等)以及含CAM类型(景天酸)代谢机制的植物中,CO2的富集和RubisCO的羧化功能位于同一组织。但是它们在不同的时间进行:在夜晚,C4途径活跃,确保苹果酸代谢,在白天,苹果酸代谢中释放的CO2会使C3机制变得活跃[16]。(见“长生草:植物适应环境限制的示例”)。
6. 不断变化的环境中的光合作用
6.1. 代谢类型如何促进植物适应环境变化?

[来源:Lars Plougmann / CC BY-SA 2.0]
温度是一种重要的环境因子,并且对C3和C4植物的光合作用具有不同的影响。例如,温度高于30℃的环境,更利于本就适合生存在极为干燥环境下的C4植物和CAM植物生长。
在强光照、高温、且水分和养料充足的条件下,C4植物几乎没有光呼吸活性,会比C3植物更高效地固定大气中的二氧化碳。例如,地球上5%的C4植物可以固定全球30%的二氧化碳。
- 而且,要产生相同的的生物量,C4植物的袖型叶片结构可使其节省三分之一的用水量。生产1kg的玉米(C4植物,图10)面粉只需要350升水,而生产1kg的小麦(C3植物,图11)面粉却需要500升水。
- C4植物氮动员要比C3植物少,因为PEP羧化酶使富含氮的RubisCO酶含量减少,以达到与C3植物相同的光合速率。

[来源:Lars Plougmann / CC BY-SA 2.0]
然而在光照和温度都较低的温带地区,C4植物的光合能力差异逐渐减弱。
此外,如果大气中的二氧化碳浓度像目前观测到的那样继续升高(见“人类活动破坏了碳循环”),在温度适宜的条件下,C3植物的光合作用有望接近C4植物。
6.2. 未来如何?
观察显示,在未来的几十年中,植物为了适应不断变化的环境很可能会获得新的机制。
通过深入了解植物为适应环境变化所采取的不同机制,我们能够研究如何培育出更好适应二氧化碳含量、温度及水环境等变化的植物。但在目前在研的众多项目中,尚不清楚哪些将有利和适用于大规模农业和工业应用。在“进化的光合作用”中指明了一些可能的方向。
7. 要记住的信息
- 通过光合作用,植物和一些特定的细菌将部分太阳转化为稳定的化学能并且同时固定了二氧化碳,从而生成了生命发育所需的重要有机物分子。
- 放射性14C用作分子标记物以及相应分析技术的发展,使破译碳代谢途径和揭示本森-巴萨姆-卡尔文循环成为可能。该循环确保了CO2碳受体的再生以及光合细胞不断改进和行使功能所必需物质(糖类,蛋白质和脂类)的合成。
- 数十亿年来,二氧化碳的碳固定整合了本森-巴萨姆-卡尔文循环,并由光合作用特定酶——二磷酸核酮糖羧化酶(RuBP羧化酶)催化。
- 由于地球氧气浓度的增加(在大气和海洋中),RuBP羧化酶也可以固定氧气,不仅具有羧化酶功能,还具备加氧功能,因此被称为RubisCO。
- 加氧功能负责合成磷酸甘油酸分子,是本森-巴萨姆-卡尔文循环的强大抑制剂。进化中的植物保留下一种通过排放二氧化碳而消除2P-乙醇酸毒性的代谢途径,即光呼吸循环。
- 其它的光合作用生物通过形成一些额外的机制发展了独特的并有效的策略,例如通过C4循环确保RubisCO附近的富含二氧化碳环境,促进其羧化作用而抑制光合作用的加氧活性。
参考资料及说明
感谢Dunod和 QUAE杂志授权再版这篇文章。
封面图片:[来源:©Jean-François Morot-Gaudry]
[1] 碳11(11C)是碳的同位素,半衰期为38分钟。因此,使用这种放射性同位素的实验必须非常短,因为它在几个小时后就检测不到了。它通常被用来在“正电子发射断层扫描”中标记分子。
[2] Benson, A.A. (1951) Identification of ribulose in 14CO2 photosynthesis products. Am. Chem. Soc. 73:2971-2972.
[3] Bassham, J. A., Benson, A. A., Kay, L. D., Harris, A. Z., Wilson, A. T. & Calvin, M. (1954) The path of carbon in photosynthesis. XXI. The cyclic regeneration of carbon dioxide acceptor. J.-Am. Chem. Soc. 76:1760-1770;
[4] 生物学是基于碳的化学,因为生物学研究中的电化学势,就是化学研究中化学能与电能的相互转化。它们能够同时维持四种不同的化学键,从而增加原子和分子各种组合的可能性,也增加了对生命多元进化与发展至关重要的有机分子多样性。
[5] 道尔顿(Dalton)是一个标准的测量单位,用来表示原子和分子的质量。最初被定义为碳12原子质量的1/12。由于分子的大小,千道尔顿(kDa)更多地用于生物学和生物化学中。大多数细胞分子的质量通常在20-100kDa之间。
[6] 氮是氨基酸和蛋白质的主要元素(大约占蛋白质质量的6%)。
[7] 奥托海因里希·沃伯格(1883-1970),德国内科医生、生理学家和生物化学家。获得1931年诺贝尔生理学和医学奖,“因为他发现了呼吸酶的性质和作用模式”。
[8] 目前,大气中的二氧化碳含量已超过400ppm(04%)。2019年,夏威夷的莫纳洛亚天文台记录到了超过415ppm的二氧化碳含量值。
[9] Lorimer G.H. (1981). The carboxylation and oxygenation of ribulose 1,5-bisphosphate: The primary events in photosynthesis and photorespiration. Annu.Rev. Plant Physiol. 32: 349-383.
[10] Tolbert N.D. (1997). The C2 oxidative photosynthetic carbon cycle. Annu.Rev. Plant Physiol. Plant Mol. Biol. 48: 1-25.
[11] Hagemann M., Kern R., Maurino V.G., Hanson D.T., Weber A.P.M., Sage R.F. & Bauwe H. (2016) Evolution of photorespiration from cyanobacteria to land plants, considering protein phylogenies and acquisition of carbon concentrating mechanisms. J.Exp. Bot., 67:2963-2976.
[12] Erb T.J. & Zarzycki J. (2018) A short history of RubisCO: the rise and fall (?) of Nature’s predominant CO2 fixing enzyme. Curr.Opinions. Biotechnology, 49:100-107
[13] Badger M.R., Price, G.D., Long B.M. & Woodger F.J. (2006). The environmental plasticity and ecological genomics of the cyanobacterial CO2 concentrating mechanism. J.Exp. Bot. 57: 249-265.
[14] 草酰乙酸盐迅速还原为苹果酸盐,并迁移到血管周鞘的叶绿体中。
[15] Christin P.A. & Osborne C.P. (2014) The evolutionary ecology of C4 plants. New Phytol. 204(4):765-781; Hatch M.D. & Slack C.R. (1970). Photosynthetic CO2-fixation pathways. Ann. Rev. Plant Physiol. 21: 141-162;
[16] Koteyeva N.K., Voznesenskaya E.V., BerryJ.O., Asaph B., Cousins A.B. & Edwards G.E. (2016) Synthesis along longitudinal leaf gradients in Bienertia sinuspersici and Suaeda aralocaspica (Chenopodiaceae). J.Exp. Bot. 67 (9): 2587-2601.
环境百科全书由环境和能源百科全书协会出版 (www.a3e.fr),该协会与格勒诺布尔阿尔卑斯大学和格勒诺布尔INP有合同关系,并由法国科学院赞助。
引用这篇文章: MOROT-GAUDRY Jean-François, JOYARD Jacques (2024年3月14日), 光合作用的碳代谢途径, 环境百科全书,咨询于 2025年4月2日 [在线ISSN 2555-0950]网址: https://www.encyclopedie-environnement.org/zh/vivant-zh/path-carbon-photosynthesis/.
环境百科全书中的文章是根据知识共享BY-NC-SA许可条款提供的,该许可授权复制的条件是:引用来源,不作商业使用,共享相同的初始条件,并且在每次重复使用或分发时复制知识共享BY-NC-SA许可声明。