植物是如何忍受含盐环境的?
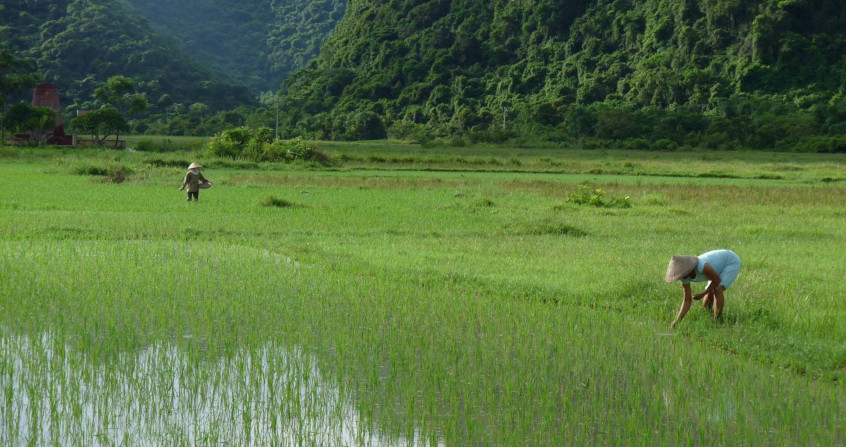
土壤受到诸如海浪冲刷等影响时会自然盐碱化。但盐碱化也可能是人类活动的结果。后一种盐碱化影响了全世界2.6亿公顷灌溉土地中的20%至30%。尽管大量的钠离子具有细胞毒性,许多植物仍然能够在盐碱条件下(例如海边)自然生长。然而,大部分的植物,尤其是农作物,如水稻——对土壤中过量的盐敏感。这是一个受到国际研究关注的食品安全问题。经过几十年的研究,人们对植物的盐度毒性和适应机制有了很好的了解。现在,这一知识被适时地运用,以获得更加耐受土壤盐分的水稻新品种。为何不把盐水灌溉谷物作为一个法宝呢!
1.植物与盐分
1.1.耐性植物
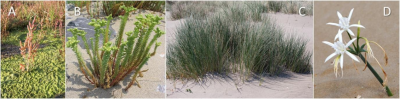
海浪飞沫由悬浮在空气中的海水水滴组成,它喷洒在海岸的植被上,使其生长的土壤盐碱化。这些植物被称为“嗜盐”,意思是“喜欢盐”。[1]“盐生植物”是指生长在异常高浓度盐分环境中的植物。例如生长在海岸、沙漠、盐沼或盐湖中的植物。
在盐沼中生长着海蓬子属植物(Salicornia sp.),其中包括大约30种可食用的物种,在沙丘上还可以找到海刺(Ephorbia paralias)、沙发草(Elymus farctus)或壮观的海百合(Pancratium maritimum)。
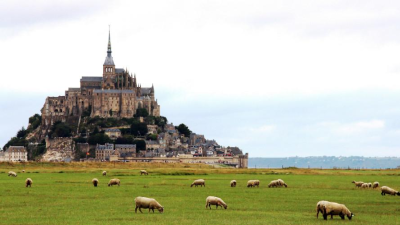
在其他纬度地区,如潮湿的热带和赤道气候区,红树林植被在微咸水中生根,说明了嗜盐草或灌木的耐盐能力。
盐生植物可以耐受500mM至1M范围内的盐浓度,从而提高了钠离子Na+毒性管理机制的有效性[2]。一些植物,如欧洲海蓬子,需要这种离子才能生长,它们被称为严格的盐生植物。
这些与众不同的的生态系统适用于发展农业;例如,盐碱草甸牧区(图2)这一农业实践,用适应土壤盐分、洪水和干旱的嗜盐植物饲养绵羊。这些植物包括海碱茅(Puccinellia maritima,一种草)、海韭菜(Triglochin maritima)或马齿合淀藜(Halimione portulacoïdes)。
1.2.敏感植物

虽然盐生植物可以在盐渍土中生长,但其他不适应这些极端条件的植物却无法做到这一点。它们是在无盐环境中生长的“甜土植物”或“糖生植物”。植物对盐胁迫具有广泛的耐受性。例如,在谷类作物中,水稻(Oryza sativa)最敏感(图3),其次是硬粒小麦(Triticum turgidum ssp.durum)和面包小麦(Triticum aestivum),大麦(Hordeum vulgare)是最具耐受性的[3]。
在盐渍土中,非嗜盐植物的生长和发育会受到影响。这是由于土壤中存在过量的可溶性盐,主要是钠离子(Na+)。盐害的可视症状是叶尖失绿,随后是叶焦、褐变和叶片死亡。这会导致植物生长迟缓、根系发育不良、不育以及种子产量减少。
土壤盐碱化的蔓延是一个重大的环境问题:每年,全世界有1000万公顷的农田被土壤盐碱化破坏。气候变化、过度使用地下水、劣质灌溉水的滥用、在半干旱至干旱气候区进行大规模灌溉以及土壤淋溶的缺乏都会加剧土壤盐碱化现象(见“土壤盐碱化”)。几十年来,植物生物学研究使人们更好地了解与盐分相关的毒性机制以及使植物能够适应盐分的机制(参见“植物生物技术和作物耐盐性”)。
2.钠离子Na+对植物细胞的毒性
土壤中高浓度的钠离子会对植物的生长发育产生一系列有害的影响:
一方面,土壤溶液中的高浓度盐会增加其水势[4];进而破坏植物通过根部获取的水分和营养。
另一方面,植物无法阻止钠离子长期进入其根细胞并转移到地上部分,导致大量的细胞中毒。
因此,一个两相模型可用来解释过量钠离子造成的有害机制:
前期效应与外部渗透压增加有关;
后期效应与细胞内钠离子的积累有关。
2.1.水、盐和水势
土壤中的盐会影响其水势。这种势能是在土壤中释放1g水所必须施加的能量。水势总是负的,并且水势越低,水和土壤之间的结合就越强[5]。纯水的水势为0;但土壤中的水不是纯净的,含有溶质,溶质是土壤中水势下降的原因。因此,土壤水势被定义如下:
根据其含水量来定义。水分充足土壤的水势值约为-0.1 兆帕,而干燥土壤的水势值约为-1 兆帕。
根据土壤中的溶质浓度来定义。在被150 mM NaCl盐溶液污染的土壤中,该值可达到约-0.4 兆帕。
为了理解这些物理化学参数在盐度问题中的重要性,必须考虑根细胞的水势。在正常条件下,根细胞的水势值约为-0.5兆帕。
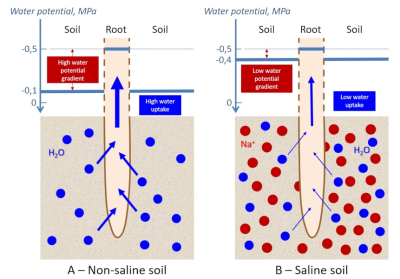
水的运动从最高电位到最低电位(换句话说,从最小负电位到最大负电位)。土壤和植物细胞之间的水势差(0.4兆帕)将允许水分从土壤(-0.1兆帕)流向到根细胞(-0.5兆帕)(图4A)[6]。
当土壤被150 mM NaCl盐溶液污染时,水势差减小至0.1 MPa(图4B)。水势差是水流过细胞膜的驱动力之一。可以估计,除了细胞对盐度的适应性反应外,这种驱动力在盐度条件下将水分从土壤转移到根系内部的效率是正常条件下的4倍!
在极端情况下,土壤含盐量会更高,理论上可以看到水分从根细胞进入盐渍土,植物通过根系脱水!这种现象让人联想到水合应力,水合应力可能有多种诱导因素(干旱、霜冻等),当土壤无法向根部提供足够的液态水以确保叶片组织的水合和蒸发时,就会出现水合应力(见“植物的固定寿命及其限制”)。
盐分的渗透作用所引起的损害不仅影响细胞的膨胀性,而且诱发会类似于水分胁迫引起的代谢变化。例如,渗透胁迫对植物的生长速率有直接影响。
2.2.盐度、光合作用和氧化胁迫
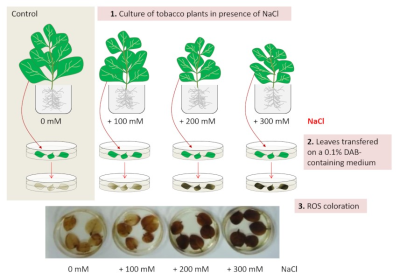
除了遭受渗透胁迫,使根细胞无法正常吸收水分之外,植物还必须应对其叶片部分的生理紊乱:
由于气孔关闭(由脱落酸激素控制的现象)和二氧化碳固定的抑制,光合作用发生改变;
电子通过光系统II的线性转移被抑制,光系统I内电子的循环转移被激活;
为了以荧光形式排出多余光能而建立的非光化学猝灭保护机制被加强[7](参见“Z-光合作用”)。
光合作用紊乱的直接后果是产生活性氧(ROS)和表达参与氧化应激管理的酶,以防止对光系统、脂质、蛋白质和核酸的损害。然而,其中一种活性氧——过氧化氢(H2O2)在耐盐环境中也具有细胞信号作用。因此,活性氧的产生、酶对活性氧的消除和细胞信号传递所需的足够数量的活性氧之间存在一种协调机制(图5)[8]。
2.3.为什么钠离子Na+有毒

图中The massive entry of Na+ in the cytosol induces plasma membrane depolarisation 钠离子Na+大量进入细胞质引起质膜去极化;Salinity enhances competition between Na+&K+ 盐度增强了钠离子Na+与钾离子K+之间的竞争;K+deficiency 钾离子K+缺乏;Na+ competes with K+ for binding to proteins,without performing the same functions 钠离子Na+与钾离子K+竞争与蛋白质的结合,而具有不同的功能;Depolarisation induces a spectacular augmentation of K+ leaking outside the cell去极化诱导细胞外钾离子K+大量渗漏;Aquaporins (water channels) are involved in Na+ entry within the cell 水通道蛋白(水通道)参与细胞内钠离子Na+的进入。
钠离子Na+的特殊毒性可能是由于其物理化学性质接近于K+(钾)。在所有生物中,钾K+是细胞质中的主要无机阳离子,其浓度(约0.1 M)通常比Na+高几倍。它在植物生理学中起着至关重要的作用。由于其细胞内的丰富性,它是蛋白质和核酸负电荷的主要无机反离子,还具有激活50多种酶反应的功能[9](参见“钾和钠:异卵双胞胎”)。
在盐环境中,Na+大量进入植物的细胞质会导致一系列反应(图6)[10]。
钠离子Na+与钾离子K+竞争根细胞对钾离子K+的吸收,因为两种离子通过几个相同的转运系统(NSCC型非选择性阳离子通道和HKT高亲和力转运蛋白)跨质膜进行转运。这种现象在盐胁迫情况下会加剧。
钠离子Na+对细胞表面具有有害影响,因为它严重破坏了质膜的电极化。这种去极化通过由其激活的钾离子K+通道(称为KOR的通道),导致细胞外的钾离子K+渗漏显著增加。
钠离子Na+与钾离子K+竞争,与重要的蛋白质结合,而不执行与后者相同的功能。因此,细胞质中过量的钠离子会抑制许多酶反应的活性,从而导致细胞功能障碍,例如影响植物的光合活性。
近期的数据表明,质膜上已知功能主要为运输水分和中性溶质的水通道蛋白(水通道)也参与了钠离子Na+进入细胞的过程!
因此,尽管土壤中存在钾离子K+,但可以说盐分依然能导致植物缺乏这种养分!
3.植物耐盐机制
3.1.什么是盐胁迫?
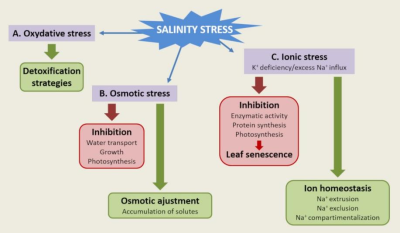
植物已经发展出多种生物化学和分子机制来抵抗土壤盐分的不利影响。盐胁迫的成分可分为三类[11]:
盐胁迫期间遇到的氧化胁迫必须通过保护和损伤修复机制在细胞水平上进行管理。
对渗透胁迫的反应使植物通过兼容溶质的生物合成和水通道蛋白(水通道)来维持水的动态平衡。
这些机制涉及钠离子Na+和/或钾离子K+转运系统的功能和调节,这些转运系统参与了对离子胁迫的响应(图7)[12]。
3.2.针对氧化胁迫的解毒策略
在玉米幼苗中,盐胁迫引起的氧化胁迫主要发生在成熟的根和叶中,幼叶中的氧化胁迫程度较小(图7A)。各种解毒策略随之而来:
H2O2含量和细胞膜氧化损伤标志物(电解质渗漏和脂质过氧化)增加。
抗氧化分子(多酚、类黄酮、抗坏血酸等)和抗氧化酶活性(过氧化氢酶、超氧化物歧化酶、过氧化物酶)在细胞中积累。
通过这种方式,ROS保护机制可以在整个植物中被激活,就像在许多其他胁迫情况下一样(参见“植物如何应对高山胁迫?”)。
3.3.如何保持水的稳态?
维持植物组织中的水分平衡(也称为水分动态平衡)对植物的生长和发育至关重要。水分通过气孔的蒸腾作用流失,通过根系吸收获得。因此,水的动态平衡由水的供应来保证,但也由植物细胞保持水分的能力来保证。
在渗透胁迫条件下,水分稳态受到干扰(见图4),植物细胞会在细胞质中积累相容的溶质以平衡渗透压(图7),如蔗糖、脯氨酸和甘氨酸甜菜碱。例如,脯氨酸的积累被描述为在盐胁迫下大量植物中的无毒和保护渗透压的过程。
3.4.维持离子平衡?
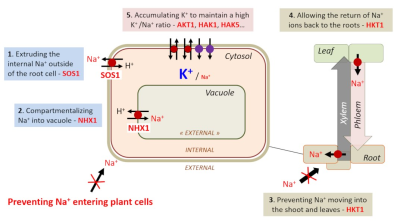
(图8 Extruding the internal Na+ outside of the root cell-SOS1 SOS1蛋白将内部钠离子Na+排出根细胞的外部;Compartmentalizing Na+ into vacuole-NHX1 NHX1蛋白将钠离子Na+区室化到液泡;Preventing Na+ entering plant cells防止钠离子Na+进入植物细胞;Preventing Na+ moving into the shoot and leaves-HKT1 HKT1蛋白防止钠离子Na+进入茎和叶中;Allowing the return of Na+ ions back to the roots-HKT1 HKT1蛋白允许钠离子Na+返回根;Accumulating K+ to maintain a high K+/Na+ ratio-ADT1,HAK1,HAK5…ADT1、HAK1、HAK5等蛋白积累钾离子K+以保持较高的钾离子K+/钠离子Na+比率)
对水稻基因组的分析已经确定了水稻耐盐基因[13]。
其中一个基因编码的钠离子Na+转运蛋白参与钾离子K+(一种必需元素)和钠离子Na+(一种有毒元素)之间的稳态。这一重大发现突出了这两种离子的转运系统在耐盐现象中的重要性。
对于能够耐受盐胁迫的植物,重要的是根细胞质中的钾离子K+/钠离子Na+比率较高,因此这些细胞含有极少量钠离子Na+(图7C)。植物通过不同的策略来实现这一目标;例如促进钠离子Na+和钾离子K+稳态的运输系统(图8)。
将多余的钠离子Na+从根表皮细胞中排出。SOS1蛋白[14]在这一过程中发挥作用,它是迄今为止鉴定到的唯一一个定位于植物质膜上的局部钠离子Na+外流系统。
一旦钠离子Na+进入细胞质,通过NHX1蛋白的活性,可将钠离子Na+区室化到液泡[15]。
防止钠离子Na+转移到地上部分。该作用由HKT1家族的选择性钠内流转运蛋白完成。该蛋白定位于根木质部薄壁组织中,通过将钠离子保留在木质部薄壁组织细胞中的过程,达到将钠离子从木质部汁液中排出的目的。耐盐(Saltol)基因座携带着编码这种运输系统的基因。
允许钠离子Na+返回根部。HKT1也在叶片韧皮部导管附近的细胞中;这将允许钠离子Na+从地上部分再循环,将钠离子Na+装载到韧皮部以进行回流。
通过钾离子K+转运系统的参与对抗钠离子Na+的毒性作用。这些系统有助于维持较高的细胞溶质钾离子K+/钠离子Na+比率。例如,在水稻中,分别编码钾离子K+转运蛋白的AKT1、HAK1和HAK5基因的个体突变导致突变植株对盐胁迫的更高敏感性。这表明钾离子K+营养在土壤耐盐性中起主要作用(图8)。
4.钠对植物可以是有用的!
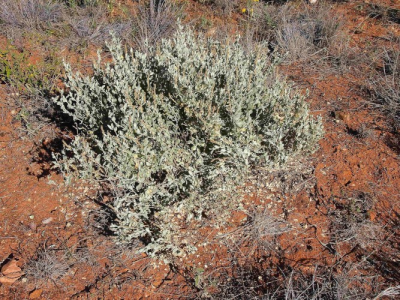
尽管钠离子Na+对植物存在毒性,它同时也是一种营养素,当钾离子K+在土壤中浓度较低时尤为如此。
土壤中的钾离子K+浓度在毫摩尔范围内时,植物可以实现最佳生长。土壤溶液中,由土壤颗粒和粘土缓慢释放的钾离子K+数量往往限制了大多数自然生态系统中植物的最佳生长。当土壤中的钾离子K+浓度非常低(微摩尔量级)时,钠离子Na+可以在某些重要功能中替代它,例如钾离子K+作为溶质维持细胞中的渗透压的功能(参见“钾和钠:异卵双胞胎!”)。
大约90%的钾离子K+储存在液泡中,并在其中发挥渗透作用。在液泡中,钠离子Na+也能起到同样的作用;然后,细胞在细胞质中动员钾离子K+,并在其中发挥代谢作用。当土壤中的钾离子K+浓度较低时,专门表示的钠离子Na+转运系统将使植物吸收钠离子Na+,以实现这一有益用途[16]。因此,钠离子Na+可以在低浓度下刺激植物生长。
嗜盐植物可能需要一定浓度的NaCl才能正常生长,如小叶滨藜(一种澳大利亚灌木,与法国盐碱草甸中发现的马齿合滨藜(Halimione portulacoïdes)密切相关,见图9)、紫穗稗(Echinochloa utilis,一种日本小米)或大花马齿苋(Portulaca grandiflora,一种马齿苋)。
5.要点
-在世界范围内,有20%的灌溉土地受到土壤盐碱化的威胁:
-具有农艺价值的植物对土壤盐碱的耐受性很低或根本没有耐受性;
-只有嗜盐植被适合在盐碱条件下生长。
钠离子(Na+)是盐干扰引起毒性的主要原因:
-根系吸收水分和养分;
-叶片进行光合作用;
-但也通过积累活性氧导致氧化胁迫。
由于其相似的物理化学性质,钠离子Na+和钾离子K+竞争,后者是植物的主要营养元素。
植物通过几个步骤对钠离子Na+的存在作出反应:
-通过保护自己免受氧化胁迫;
-通过积累溶质来抵消土壤中过多钠离子Na+的渗透效应;
-通过限制钠离子Na+在根中的吸收,增加其在根细胞外的排出,将其限制在液泡内,并管理其从叶片的运输和排除。这种植物还改善了植物的钾营养。
参考资料和说明
封面图片:吉婆岛(Cat Bá)的稻田(越南)。[来源:©Doan Trung Luu]
[1] 嗜盐植物也被称为嗜盐植物,与在无盐环境中生长的嗜糖或“糖生”植物相反。
[2] Flowers T.J., Galal H.K., & Bromham L. (2010). Evolution of halophytes: multiple origins of salt tolerance in land plants.Funct. Plant Biol. 37, 604-612. doi: 10.1071/FP09269
[3] Munns R. & Tester M. (2008) Mechanisms of Salinity Tolerance. Ann. Review Plant Biol. 59: 651-681
[5] 以压力单位表示,如帕斯卡(Pa)
[6] Rengasamy P.,North S.和Smith A.(2010)莫里灌溉区土壤和水中碱度和盐度的诊断和管理。南澳大利亚州阿得雷德大学。
[7] Stepien P. & Johnson G.N. (2009) Contrasting Responses of Photosynthesis to Salt Stress in the Glycophyte Arabidopsis andthe Halophyte Thellungiella : Role of the Plastid Terminal Oxidase as an Alternative Electron Sink. Plant Physiology 149 (2)1154-1165; DOI: 10.1104/pp.108.132407
[8] Yadav N.S., Shukla P.S., Jha A. et al. (2012) The SbSOS1 gene from the extreme halophyte Salicornia brachiata enhances Na+ loading in xylem and confers salt tolerance in transgenic tobacco. BMC Plant Biol 12, 188. https://doi. org/10.1186/1471-2229-12-188
[9] Bhandal I.S. & Malik C.P. (1988) Potassium estimation, uptake, and its role in the physiology and metabolism of floweringEncyclopédie de l’environnement11/11Généré le 26/10/2021plants. Int. Rev. Cytol. 110: 205-254
[10] The general outlines of this article are original, but inspired by many pedagogical schemes. The most emblematic of them is“Teaching tools in Plant Biology (2014) Plant Nutrition 1: Membrane transport and Energetics, Potassium nutrition, and Sodiumtoxicity. DOI: https://doi.org/10.1105/tpc.114.tt0914.”
[11] Munns R. & Tester M. (2008) Mechanisms of Salinity Tolerance. Annu. Rev. Plant Biol. 59, 651-681.
[12] 植物营养1:膜运输和能量学、钾营养和钠毒性
[13] 这种基因被称为Saltol(耐盐)。它编码HKT1家族的选择性钠内流转运体。水稻基因组在2005年被完全测序。它的基因库大约有37500个,目前都可用。任何可见和可测量的农艺性状(表型),如耐旱性、耐盐性或株高,都可能与一组基因(基因型)有关。
[14] SOS1对盐过度敏感;这是一个Na+/H+反端口。反转运蛋白是一种膜蛋白,参与不同离子沿相反方向穿过膜(如质膜)的主动转运,其中一个方向为Na+,另一个方向为H+。
[15] 这是NHX1类型的Na+/H+反端口。
[16] Horie T., Costa A., Kim T. H., Han M.J., Horie R., Leung H.-Y., Miyao A., Hirochika H., An G. & Schroeder J.I., (2007)Rice OsHKT2;1 transport mediates large Na+ influx component into K+-starved roots for growth. EMBO J. 26, 3003-3014.
环境百科全书由环境和能源百科全书协会出版 (www.a3e.fr),该协会与格勒诺布尔阿尔卑斯大学和格勒诺布尔INP有合同关系,并由法国科学院赞助。
引用这篇文章: LUU Doan Trung (2024年3月14日), 植物是如何忍受含盐环境的?, 环境百科全书,咨询于 2025年3月30日 [在线ISSN 2555-0950]网址: https://www.encyclopedie-environnement.org/zh/vivant-zh/how-plants-tolerate-salty-diet/.
环境百科全书中的文章是根据知识共享BY-NC-SA许可条款提供的,该许可授权复制的条件是:引用来源,不作商业使用,共享相同的初始条件,并且在每次重复使用或分发时复制知识共享BY-NC-SA许可声明。