光、视觉和生物钟
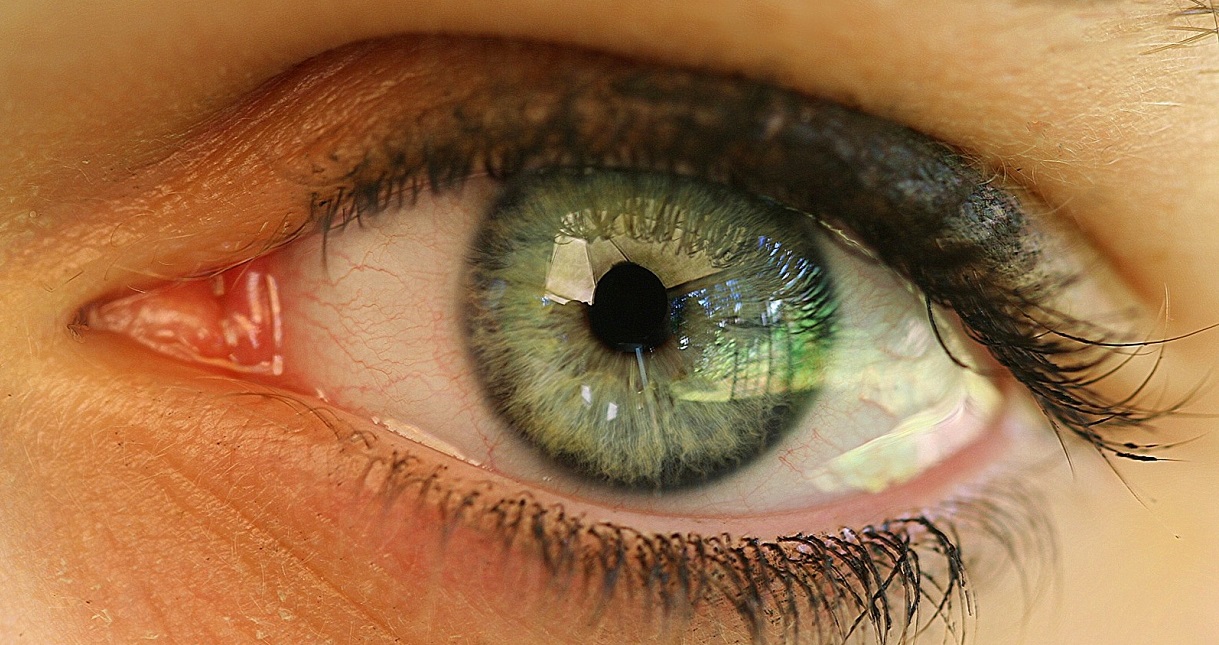
地球生命自诞生以来,就受到各种力量的选择而不断演化,其中以太阳光的作用最为重要。光及其自然周期性,包含日变化和季节性变化,使得生物体能感知它们所处的时空环境。光及其自然周期性如何使生物体适应其生存环境?动物界出现过哪些视觉和非视觉光受体系统?视觉和生物钟系统是如何使生物控制其环境、预测环境的变化,因而从中获益的?
我们的恒星太阳有46亿年的历史,是一个真正的核反应堆:每秒钟在其核心有6.2亿吨氢气被转化为6.16亿吨氦气,损失的四百万吨质量被转化为向外辐射的能量,即光子,换言之即向外发光。这些光子在以30万千米/秒的速度运行8分钟后到达地球,并以每秒每平方毫米6 400万亿个光子的通量进入地球大气层,其中一些被大气中的原子和分子吸收或反射回太空,剩余部分到达地表,照亮我们的星球。
地球自从38亿年前出现生命以来,已经经历了14 000亿次昼夜循环和38亿次季节循环(见焦点1:光的周期性与生物)。因此,随着光敏系统、视觉和非视觉系统以及生物钟的出现,光对生命的演化历程产生了强烈的影响。换言之,光及其自然循环使生物体能够感知并且适应其时空环境[1]。
1.光感受的演化史
1.1. 感光蛋白
从最早出现的感光蛋白到现在复杂的视觉系统,感光系统的进化经历了不同的阶段,在此过程中有一系列标志性事件。生物光感受起源于一类重要的光敏蛋白——视蛋白,该类蛋白有两个超家族,分别是I型和II型视蛋白[2]。I型视蛋白,也称为微生物视蛋白,在生物界的三域(古细菌、细菌和真核生物)中都有分布。该蛋白的主要功能是调节生物体对光的定向反应或趋光性,以及吸收光产生能量。I型视蛋白是什么时候出现的呢?由于它们在所有生物体内都普遍分布,因而可以判定其在生物界的三域分离之前就已经产生了。这种蛋白起源久远,证明了光对生命具有极端重要性。II型视蛋白,也称动物视蛋白,仅存在于高等真核生物中,主要调节视觉光感受和生物钟。这类视蛋白的先祖7亿年前就出现在原始后生动物中了,这是第一种可自由活动的异养多细胞生物。这两种视蛋白在结构上有类似之处,但两个超家族之间没有系统发育联系。相反,它们是各自独立进化而来的[2]。
1.2. 感光细胞
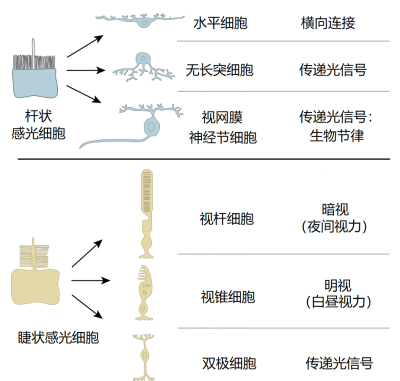
6亿年前,随着第一个后生动物及II型视蛋白的出现,地球上有了专门的感光细胞。然而,直到5.4亿年前的寒武纪,经过快速的辐射进化,才出现了首个复杂的视觉和非视觉系统。在无脊椎动物和脊椎动物中,有两类专门的感光细胞,称为杆状感光细胞和睫状感光细胞,它们的顶端表面分别具有微绒毛和纤毛(见图1)。在脊椎动物中,杆状感光细胞可分化形成参与生物钟控制的光敏感性神经节细胞。睫状感光细胞则可分化为视锥细胞和视杆细胞,分别参与控制明视(昼视)和暗视(夜视)[3](图1)。
1.3 非视觉和视觉系统
时至今日,大多数动物都具有复杂的非视觉和视觉系统。 在非视觉系统中,我们可以区分不同的结构:
- 位于头颅内的小苹果形状的器官:松果体。除哺乳动物外,所有脊椎动物的松果体都具有光敏性;
- 与松果体有联系的器官,因此它的名称为松果旁体,鱼的这个器官在颅内;
- 两栖动物和爬行动物的额器或顶眼;
- 除哺乳动物外的所有脊椎动物都有的脑深部光受体;
- 皮肤光受体,存在于几种脊椎动物和无脊椎动物的皮肤上,主要是两栖动物和头足类动物。
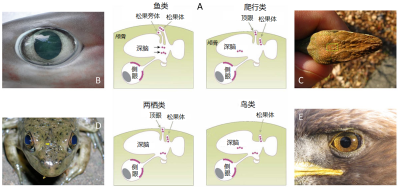
A. 剖面示意图,红色圆圈表示感光细胞(根据福斯特和索尼 [5]修改后的图 [4] )。B. 鲨鱼的侧眼(大眼六鳃鲨)[©照片 让-卢·贾斯汀(来源:维基百科)];C. 爬行动物(安乐蜥)头顶的顶眼(在矩形的中心);[©照片 阿尔法之狼(来源:维基百科)];D. 牛蛙(北美牛蛙)的侧眼和顶眼(两只眼睛之间的灰色小圆圈,由黄色箭头表示)[©照片 阿尔法之狼(来源:维基百科)];E. 雕的侧眼(金雕)[©照片 彼得·卡明斯基(来源:维基百科)]。
在这些生物中,侧眼提供视觉信息,因此参与了视觉感知——感知光线对比度和颜色。哺乳动物的侧眼也参与接收非视觉信息,并在生物钟的调节方面发挥重要作用[5]。
这些器官都参与非视觉光感知,并在生物钟的调节和控制中发挥重要作用(图2)。
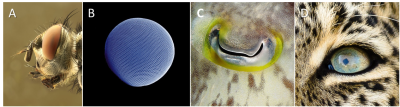
复眼(A. 带有复眼的丽蝇科苍蝇头部[©照片来源:慕尼黑的理查德·巴茨,又名马克罗·弗莱克,维基百科];B. 南极磷虾[©照片来源:格尔德·阿尔贝蒂和乌韦·基尔斯,维基百科])和单眼(C. 乌贼属[©照片来源:亚历山大·瓦舍宁,维基百科)];D. 美洲豹[©照片来源:雅克·乔亚德] )
视觉系统指的是大多数无脊椎动物和所有脊椎动物有的侧眼。有十种结构不同的类型。其中最引人注意的是昆虫的复眼和脊椎动物和软体动物的单眼(图3)。
2.视觉
所有脊椎动物侧眼的结构都相同,类似于单镜头相机:光线通过瞳孔(镜头)在眼睛后部的视网膜(敏感面)上形成倒像。视网膜由五种相连的神经元组成:即感光细胞、水平细胞、双极细胞、无长突细胞(无轴突)和神经节细胞(图4)。最常见的区别是有些物种的视网膜感光细胞在内部,另一些在外部[5](图4)。
感光细胞包括视锥细胞和视杆细胞。前者对强光照敏感,参与称作明视的日间视觉;后者仅对弱光产生响应,参与的是称为暗视的夜间视觉。这些感光细胞在物种内以及不同物种之间多样性都非常高,但是实际上每种视锥细胞和视杆细胞都只含有一种特定的感光蛋白,只对特定波长的电磁波谱敏感。因此,每一种动物都有其独特的单色或多色视觉(见下文)。鱼类、两栖动物、爬行动物和鸟类在很大程度上是四色生物:它们通过四种感光细胞处理视觉信息。哺乳动物通常是二色生物,这很可能是与其进化史中长期占据夜间生态位有关。一些灵长类动物,如人类,则是一个明显的例外,他们是三色生物[3]。对哺乳动物而言的这一新特性是由一个基因重复产生的(见焦点2:哺乳动物和灵长类动物色觉的进化)。

在哺乳动物中,构成光的光子被感光细胞、视锥细胞(C)和视杆细胞 (Bt)以及非视觉光敏感性神经节细胞(pRGC)吸收,感知到的信息随后通过视神经传递到外侧膝状体处理获得视觉感光,同时传递到视交叉上核(生物钟)处理得到非视觉感光。位于视网膜中的水平细胞(H)、双极细胞(B)、神经节细胞(G)和无长突细胞(A)将光信号从感光细胞传递到视神经。[来源:修改自[4],引自阿伦特[3][4]。
当视觉光受体接收到光子时,会通过双极细胞将光信号——即光子的数量——传递到视网膜的神经节细胞,形成视神经的神经节细胞的轴突将这些信息传递到外侧膝状体(膝状),通过与视皮层交流,在视皮层处翻译出光信号。在信息传递过程中,水平细胞促进光感受器之间的横向连接,并调节它们的活性以增强对光信号的敏感度。另一方面,无长突细胞使得双极细胞和神经节细胞建立联系,参与信息传递。值得注意的是,有一个光敏神经节细胞亚群将光信号直接传递到视交叉上核(位于视交叉上方),即生物钟的中心[6](图4),下文将讨论这个内容。
因此,生物体能够感知其环境发出的视觉信息,从而感知空间环境。
3.生物钟和生物节律
3.1. 生物节律和昼夜循环
“生物节律”一词指在一个生物体内中、在一段时间内规律性的周期性重复现象。特点物种的生物节律由基因决定,通过内部生物钟产生,即使没有外部刺激也保持活跃状态。生物节律可以分为以下三种类型:
– 昼夜节律,也称为日节律,周期大约为24小时;
– 短昼夜节律,节律周期短于24小时;
– 长昼夜节律,节律周期超过24小时; 接近地球年的年节律属于这一类。[6]

内部生物钟受外部信号刺激驱动并与之同步,其中最重要的是昼夜节律,给生物体带来了多种好处。与温度、湿度或潮汐的周期性等外部刺激不同,昼夜节律没有年际变化,只与环境的季节变化相关。因此,在24小时昼夜节律的驱动下,生物体可以预测环境的季节性变化,在适当的时期表现出特定的生理和行为特征,从而生存下来并从环境中受益[7](图5)。
3.2. 中央生物钟和外周生物钟
哺乳动物体内最主要的生物钟位于下丘脑的视交叉上核,由大约20 000个神经元组成,能自发地、周期性地激活“生物钟”基因。在没有外部刺激的情况下,生物钟基因的表达周期约为23~25小时,因物种而异。这些基因一旦被激活,又会控制其他基因的表达。总的来说,在哺乳动物中近20%的基因组受体内主要生物钟控制[8]。
还有次级的外周生物钟。事实上,在大多数外周组织中都有生物钟基因表达。然而,如果没有体内的主生物钟,不同外周组织中生物钟基因的表达周期将是杂乱的。因此,必须有视交叉上核来同步这些次级生物钟。
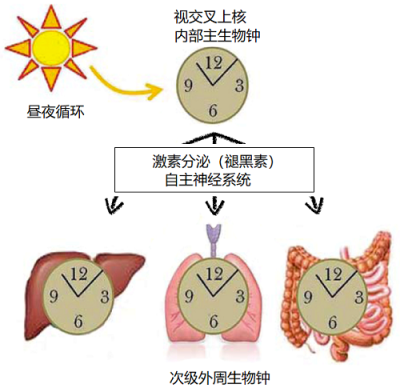
哺乳动物的内部主生物钟与24小时的昼夜周期同步,该生物钟又通过分泌激素和激活自主神经系统,使身体内的外周次要生物钟同步。[来源:根据参考图修改[9]]
内部主生物钟的周期之所以能严格保持在24小时,主要是因为地表环境有昼夜交替。哺乳动物由侧眼的视网膜感知光信号。正如我们看到的,内部生物钟由几种感光细胞组成,包括参与非视觉信息传递、对波长460~480纳米(蓝色)敏感的神经节细胞。这些细胞通过视网膜-下丘脑通路,将光信号传输到视交叉上核,然后信号激活或抑制构成视交叉上核的神经元中的生物钟基因的表达。通过这种方式,内部生物钟能够与昼夜变化同步[8]。
同步之后,视交叉上核通过分泌激素和激活自主神经系统,将其节律赋予大脑结构和次级外周生物钟。特别重要的是,视交叉上核调节松果体的活动:白天抑制而晚上刺激松果体细胞的活性。活跃的松果体细胞产生并分泌睡眠激素——褪黑素,褪黑素与位于次级外周生物钟细胞膜上的特定受体结合,向身体表明天黑了。由此可知,视交叉上核将这种昼夜节律赋予整个生物体[9]。(图6)。
4.自然光及其在生命中扮演的角色
4.1. 自然光与生态系统
在一天24小时内,生物感知到的光强度有几个量级的变化。换言之,每个小时都有特定的光照强度,因此环境被划分为循环变化的昼和夜。这种昼夜循环交替也使不同动物物种有不同的活动规律:可以分为白天活动、夜间活动、黄昏活动或昼夜活动。这种活动时间的差异具有重要的生态和演化意义[10]。值得一提的是,不仅是昼夜交替循环,季节周期和月周期也参与了动物生态位的分离和生物演化(参见焦点1:光的周期性与生物)。
环境的分时利用有利于竞争物种的共存以及被捕食者与捕食者之间的平衡。几个竞争的物种如果不是在相同的时间争夺同一资源,那么它们之间的直接冲突就会降低。同样,如果一种资源能够在两个相连的利用期之间完全再生,那么该资源就可为多个物种所用。因此,竞争者共存就得到了保证。。被捕食者与捕食者的平衡受捕食风险的时间波动及其可预测性影响,当有多个物种形成捕食关系时,不同物种可以通过活动时间分离,降低被捕食风险,同时增加对环境资源的利用[11]。例如,防御能力有限的小型哺乳动物演化出了夜间活动模式,从而降低了被捕食的风险。这样也确保了被捕食者和捕食者能共存。
对环境的分时利用和活动模式分异使物种具有不同的解剖、生理与行为特征。例如,昼行性动物利用视觉进行交流和捕食,它们的视网膜内有丰富的视锥细胞;相反,夜行性动物利用嗅觉、听觉和触觉进行交流和捕食,它们的眼睛瞳孔很大,有些动物甚至形成了一层能够反射光线的膜(反光膜),其视网膜上富含视杆细胞。由于昼夜光照强度不同,生物演化出了复杂的适应性活动模式[11]。
4.2. 自然光和生物个体
自然光及其周期性变化提供了非视觉和视觉信息,对生物体至关重要。对于任何一个物种,光所提供的光照强度或光周期性变化等非视觉信息使个体能在环境中确定自己的位置,根据光照强度梯度迁移到适宜的环境,判断季节性变化并使其生物节律同步。为适应所预测的季节变化,生物体必须具备生理和行为特征[11],如迁徙、营养储备、冬眠以及繁殖。为此,生物体必须将其生物节律与可作为“日历”的地球物理指标同步,这就是自然光和光周期性变化发挥的作用[1][11]。
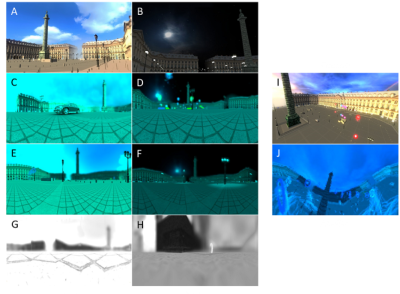
最后,对特定的物种,光所提供的对比度和颜色等视觉信息使个体能够探测到背景中的物体、定位环境中无生命或有生命的物体(图7),以及表达用于伪装、模仿或两性异型的色彩符号。因此光提供的视觉信息对于导航、觅食、捕食、交流和繁殖必不可少[1]。
总之,自然光及其周期性变化是一种重要的选择力量,通过演化,决定了生物的视觉和非视觉系统以及生物体内的生物钟。在生物个体层次,自然光及其周期性变化使生物体能够识别其所处时空环境,使它们能够控制、利用环境并预测环境的变化。在生态系统层次,自然光及其周期性变化确保了不同物种在时间和空间上对环境分割利用,是保持种内和种间平衡的关键因素。
参考资料和说明
[1] 尼尔森·DE (2009) 眼睛和视觉引导行为的进化,伦敦皇家学会哲学论文集: 生物科学364, 2833-2847;
[2] 斐纳德·R (2006) 对眼睛的进化照射遗传学的光芒,科学 313, 1914-1918
[3] 阿伦特·D (2003) 眼睛和光感受器细胞的进化,国际发育生物学杂志 47, 563-571.
[4] 乐·塔勒克T. (2013) 光、视觉和生物钟,物种9, 12–21. (法语版)
[5] 罗迪克·R (2003) 想象. 布鲁塞尔,德波克大学
[6] 寇卡利·WL & 索特恩·RB (2006) 生物节律概况. 纽约, 斯普林格出版社.
[7] 恰勒特·E (2007) 微评:昼伏夜出的哺乳动物视交叉上生物钟的传输. 内分泌学148, 5648-5655.
[8] 傅立叶·R, 福赛斯·C & 凯沙瓦兹安·A (2013) 昼夜节律紊乱:酒精对相关炎症和代谢性疾病的潜在影响,酒精研究:当前评论 35, 87-96.
[9] 迪伯纳·C, 薛伯伦·U & 阿尔布雷希特·U (2010) 哺乳动物的昼夜节律系统:中央和外围生物钟的组织和协调。生理学年度评论 72, 517-549.
[10] 克隆费尔德-肖尔·N & 达扬·T (2003) 一类生态资源时间上的分配。生态学、进化学和系统学年度评论 34, 153-181.
[11] 勃莱德肖·WE & 浩尔萨普费尔 CM (2007) 动物的光周期进化。生态学、进化学和系统学年度评论38, 1-25.
环境百科全书由环境和能源百科全书协会出版 (www.a3e.fr),该协会与格勒诺布尔阿尔卑斯大学和格勒诺布尔INP有合同关系,并由法国科学院赞助。
引用这篇文章: LE TALLEC Thomas (2024年3月11日), 光、视觉和生物钟, 环境百科全书,咨询于 2025年4月2日 [在线ISSN 2555-0950]网址: https://www.encyclopedie-environnement.org/zh/vivant-zh/light-vision-biological-clocks/.
环境百科全书中的文章是根据知识共享BY-NC-SA许可条款提供的,该许可授权复制的条件是:引用来源,不作商业使用,共享相同的初始条件,并且在每次重复使用或分发时复制知识共享BY-NC-SA许可声明。